Chapter 10 Subdural electrode implantation and recording in MRI-negative epilepsy surgery
MRI-Negative Epilepsy, ed. Elson L. So and Philippe Ryvlin. Published by Cambridge University Press. © Cambridge University Press 2015.
Introduction
Noninvasive evaluation is often sufficient when planning epilepsy surgery, and improved characterization of seizures, better understanding of epilepsy syndromes, and advances in neuroimaging in particular have made the use of intracranial electrodes less necessary than in the past. However, noninvasive testing at times fails to provide adequate information to plan surgery, and intracranial video-EEG monitoring remains a valuable tool. The view that intracranial EEG is a “gold standard” ascribes greater reliability and accuracy than it deserves, but the data provided by the EEG enable surgery to be performed when surgery would otherwise not be possible. The intracranial EEG can identify the zone in which interictal abnormalities predominate, and delineate areas in which seizures arise. When these regions are considered in light of the clinical history, examination, and other laboratory features, surgery is often successful. Intracranial EEG must delimit the margins of the epileptogenic zone (defined as the cortex necessary and sufficient to generate seizures) and the function of cortex within the bounds of that zone may need to be defined.
Hence, the anatomy and physiology of the epileptic zone dictate how the EEG is best obtained. Different types of intracranial electrodes may be used to define the cortical areas that must be excised. When buried cortex, such as hippocampus, amygdala, and gyral depths, are suspect, depth electrodes are best suited, since they penetrate the brain and record directly from these areas. When superficial neocortex is believed to contain the zone of interest, and particularly when functional mapping is required, then subdural electrodes play an essential role. Depth electrodes are discussed elsewhere in this text. The present chapter will review how subdural electrodes are used to define the extent of resection in patients with negative MRI scans. The reader should be reminded that many patients are evaluated with simultaneous use of both subdural and depth electrodes to achieve optimal sampling of cortex. We will review the strategy used when planning subdural investigations, effective use of subdural electrodes, results of subdural electrode recording, and the complications associated with their use.
Subdural electrodes
Subdural electrodes are constructed by embedding platinum discs in an inert flexible material, such as silastic. The contacts usually have an exposed diameter of 1.5–2.5 cm, though this can be customized. Commercial electrodes are often spaced 1 cm apart, but inter-electrode distances can also be customized if closer spacing is required. The contacts can be arrayed in a variety of geometric configurations though they are most commonly placed in linear arrays, known as “strip electrodes,” or rectangular arrangements, known as “grid electrodes.” For ordinary clinical purposes, subdural strip electrodes are fabricated in linear arrays of four to eight contacts, while grid electrodes come in a variety of configurations, ranging from including 2 x 2 to 8 x 8 (Figure 10.1). The number of grid electrode contacts can far exceed this, however, and is limited only by technical limits in the number of recording channels and practical size constraints. Hence, grids containing hundreds of contacts can be fabricated for research (or clinical) purposes, placing electrode contacts at small intervals. Portions of the grid can be removed if needed in the operating room to adapt the grid to local brain anatomy. In addition, the contacts need not be placed in straight lines, but can be curved to account for gyral anatomy (most often used when recording from interhemispheric cortex, i.e., pericallosal or cingulate gyri).
Figure 10.1 (a) Subdural strip electrode with connecting cable. A linear array of contacts is spaced 1 cm apart, with wires running from each contact through the silastic matrix to a cable that attaches to a connecting cable that can plug into a jackbox. (b) Subdural grid electrode in an 8 by 8 contact array. Contacts are spaced 1 cm apart, with wires running from each contact to cables that will attach to a connecting cable that plugs into the jackbox.
Figure 10.2 shows an intracranial implantation of strip and grid electrodes. Strip electrodes can be inserted via a burr hole or a craniotomy; their narrow width allows for easy insertion, whereas grid electrodes must be placed through a craniotomy because of their size. There are advantages and disadvantages to placing electrodes through a burr hole instead of a craniotomy and these differences often help decide which type of implantation is performed. Placing electrodes though a burr hole is less invasive than inserting them via a craniotomy and is associated with lower infection rates (1), probably because this method does not produce avascular segments of the skull. However, the surgeon cannot directly visualize the cortex when placing subdural strips through a burr hole to ensure that contacts are placed precisely where desired. Adhesions, veins, variations in gyral anatomy, and bony protrusions can all misdirect the subdural electrode strip, sometimes far from its desired location. Precise targeting and homotopic sampling is therefore rarely possible. When sliding along the pial surface, subdural strip electrodes can occasionally pierce the brain, if misdirected by a bony prominence or by accidentally detouring into a sulcus. Subdural electrodes are easily removed either at the bedside or in the operating room, much more easily than a subdural grid. Because strips have a lower volume than grids, they have less potential for producing mass effect and are less apt to cause herniation of the brain. Because subdural strip electrodes consist of a single row of electrode contacts, their value in defining the spatial extent of interictal and ictal EEG discharges is limited. However, this limitation can be overcome by placing several strips approximately parallel to each other. In some locations, e.g., recording from the temporal or frontal pole, one can readily ascertain the posterior extent of the resection line with a single strip electrode; a single strip directed from lateral to mesial temporal or frontal lobe cortex is adequate to determine whether seizures start in basal or lateral cortex (though the anterior to posterior extent of the discharge might not be apparent).
Figure 10.2 Skull X-ray showing an 8 x 8 cm subdural grid spanning the right frontal, parietal, and temporal lobes. Subdural strip electrodes have also been placed over occipital and posterior temporal cortex, basal, and anterior temporal cortex, and frontal lobe. This exemplifies a focused implantation with the grid, with added sampling of more distant regions to ensure that an unexpected ictal onset is not also present.
Subdural grid electrodes are placed through a craniotomy, inserted under direct visualization. They can therefore be accurately placed without the ambiguities or limitations of inserting a subdural strip electrode through a burr hole. The larger size of grid electrodes allows more comprehensive coverage of a cortical region and enhanced spatial resolution. Helpful for defining interictal and ictal discharges, subdural grids are particularly valuable for mapping eloquent cortex with electrical stimulation. As noted above, morbidity of grids is greater than subdural strips (particularly with regard to infection risk), patient discomfort is inherently greater because of the need for a craniotomy, and risk of herniation is greater (2). While electrodes can be left in place for many weeks if necessary, it is desirable to complete intracranial EEG recording quickly to reduce infection risk. Most centers leave subdural electrodes in place from 4–14 days, removing electrodes once the clinical question has been answered, and physicians should hasten the process when employing grids to minimize risk of infection.
Subdural electrodes are subject to limitations. As noted above, they are not well suited to record EEG from buried cortex, such as the hippocampus. The extensive array can restrict the placement of depth electrodes, and a remarkable amount of cortex beneath the grid lies between electrode contacts and is not sampled, particularly within the depths of sulci. A neurosurgical procedure is needed to place the electrodes, and if seizures first appear at the edge of a grid or in many contacts at once, moving the grid or adding electrodes is not a trivial matter. It requires a second operation. It is possible that the mass effect and trauma of grid insertion alters the electrophysiology of adjacent cortex, thereby affecting interictal and ictal discharges. Lastly, interpretation of the intracranial EEG is challenging, and it is often difficult to precisely determine when and where seizures start. Ictal discharges might gradually intrude into the EEG background, and be masked by changes in normal background frequencies caused by arousal. Improvements in recording techniques have revealed intracranial EEG patterns not visible in the past (e.g., high-frequency oscillations), and our understanding of EEG interpretation continues to improve as more knowledge is gained.
Recording the EEG
Commercial EEG equipment is designed to record from intracranial electrodes. Most systems will allow the neurophysiologist to record from as many channels as desired, and it is not uncommon to record from hundreds of contacts at once. We routinely record 196 channels of intracranial EEG in our institution, though properly examining so many channels can pose practical problems because of limits in the human visual system and display screens. The EEG should be sampled at 1000 Hz or 2000 Hz for accurate representation of fast frequencies and detection of high-frequency oscillations. If microwires are used to record from individual neurons, then faster sampling rates, up to 20 000 Hz are required. Synchronized video helps relate specific behaviors to the EEG. When recording intracranial EEG, it is critical to ensure that the ictal changes in the EEG precede any alteration in behavior. Should the EEG lag behind behavior, the earliest EEG discharges are certainly propagated from an area of the brain without EEG sampling; additional electrodes then need to be inserted to define the ictal onset zone.
Indications for use of intracranial electrodes
Intracranial electrodes are used when noninvasively obtained data is insufficient to recommend a safe therapeutic procedure, and it is reasonable to believe that further information will likely lead to an operation. A variety of protocols are employed, subscribing to the general principle that intracranial EEG must be performed when an epileptogenic structural lesion has not been adequately identified with noninvasive testing (Table 10.1). This situation may occur if: 1. the data are discordant, raising the possibility that seizures emanate from more than one area, or; 2. the data are insufficiently localizing to confidently define the location of the epileptogenic zone. In MRI-negative patients, any conclusion about the presence of a structural lesion is inferential since the lesion cannot be visualized, and these individuals are more likely to require intracranial EEG. An example of an MRI-negative patient with discordant data would be the circumstance in which the aura and seizure semiology suggested a left frontal lesion, but the interictal spikes emanated from the left frontal and anterior temporal regions, and positron emission tomography (PET) showed right temporal hypometabolism. An example of an MRI-negative patient with inadequate data would be one whose aura was nonspecific, interictal EEG showed left anterior temporal spikes, but seizures were nonlocalized, and PET and other noninvasive tests were normal. In contrast, the MRI-negative patient with an epigastic aura, interictal right anterior temporal spikes, right anterior temporal seizures, and right temporal hypometabolism with PET scanning with no conflicting data does not require intracranial EEG; a right anterior temporal resection can be offered with a high probability of success (3).
Intracranial electrodes, especially subdural electrodes may be implanted when the epileptogenic region has been established with reasonable certainty, but surgery poses risk of causing a neurological deficit. For example, a patient whose seizures begin with tonic posturing of the right hand and whose scalp EEG shows left frontal spikes in the interictal state, left frontal seizure onset, and focal, restricted left frontal hyperperfusion with ictal single photon emission computed tomography (SPECT or SISCOM) does not have a cryptic epileptogenic zone. It is undoubtedly located in the left frontal lobe, anterior to the primary motor cortex. However, the proximity of that zone to vital primary motor cortex means that surgery could produce a lasting paresis of the hand. In this circumstance, two options could be used. One could proceed directly to surgery, map primary motor cortex, and then excise cortex immediately anterior to motor cortex, perhaps using electrocorticography to search for interictal disturbances that might better define the epileptogenic zone. Alternatively, one might prefer to use chronic intracranial video-EEG with subdural electrodes, to better characterize the area responsible for both interictal and ictal EEG abnormalities, and then map motor function in a less pressured environment than the operating room. In the absence of a structural lesion in the MRI to help guide the resection, the latter choice – chronic intracranial EEG – might afford more confidence and reduce the risk of a lasting deficit.
Strategies for using subdural electrodes
How does one decide where to place intracranial electrodes? Positioning electrodes relies on developing a reasonable hypothesis regarding the location of the epileptogenic zone. The objective of the intracranial study is to define the epileptogenic zone as best as possible and identify eloquent cortex. An intracranial study should determine whether surgery can be performed, and if so, define the margins of the cortex to be removed and locate relevant eloquent cortex. Proper planning can occur only after acquiring a careful and detailed history, performing a neurological examination, obtaining scalp interictal and ictal EEG, MRI scan, positron emission tomography (PET), neuropsychological testing, and various other tests such as functional MRI (fMRI) or diffusion tensor imaging (DTI), single photon emission computed tomography (SPECT), and magnetoencephalography (MEG). Once these assessments are complete, plans can be made to place intracranial electrodes over or within suspect cortex to finalize a surgical plan. Electrodes cannot be placed everywhere in the brain, so it is important to have a high pretest probability of the possible location(s) of the epileptogenic zone to guide implantation of the electrodes. Where to place electrodes is determined by the presumed location of the epileptogenic zone and the location of alternative areas that might be responsible for seizures. It is often necessary to record EEG from cortical areas that are not part of the ictal onset zone but might be involved early in the course of seizure propagation since removal of this cortex might be necessary to abolish seizures in some individuals (it is part of the epileptogenic zone). Sampling from cortex outside the epileptogenic zone is also critical for defining that zone; after all, one cannot know that a particular area or lobe comprises the entirety of the epileptogenic zone unless one also records EEG from other areas in the brain and demonstrates that seizures do not start in those regions. For example, if a neurophysiologist only records intracranial EEG from the right frontal lobe, all seizures will appear to originate there irrespective of the actual location of the epileptogenic zone; seizures might have begun elsewhere and appeared in the right frontal lobe electrode contacts only after propagating there from the primary site. In addition, assessing the routes and timing of seizure propagation can aid in determining whether the presumed ictal onset zone is a plausible one. For example, if ictal onset appears in a frontal lobe and the earliest site of seizure propagation is located in the contralateral occipital lobe, one can be reasonably confident that the presumed frontal localization is incorrect, given the lack of direct connections between these lobes; patterns of seizure spread should comport with established brain anatomic connections. Lastly, if the epileptogenic zone is presumed to be located near functional cortex, then electrode contacts will need to be placed over a broad enough area to ensure that eloquent cortex can be defined.
In MRI-negative epilepsy, how might one proceed in planning an intracranial investigation? The key steps are summarized in Table 10.2. The history is particularly important. While many auras or early signs are not well localizing, others can be quite specific and suggest the location of the epileptogenic zone (4, 5). For example, a seizure beginning with bilateral proximal limb movements and preserved consciousness suggests supplementary motor cortex localization, throat tightening might suggest frontal opercular localization, a formed visual or auditory hallucination may indicate occipital or temporal association cortex, an epigastric aura and early oral automatisms suggest mesial temporal localization, and so forth. Lateralized motor features, such as versive head and eye deviation, focal clonic or tonic movements, and the sign of four at secondary generalization of complex partial seizures aid in identifying the hemisphere from which seizures emanate. The symptoms may or may not contain lateralizing features, but these symptoms and signs are nonetheless useful signposts on the road to localizing the epileptic focus, and are particularly helpful when considered in concert with other diagnostic tests. A particular constellation of clinical features might suggest that a patient has a specific epilepsy syndrome, which may also aid in localization. Hence, the entire clinical picture must be considered and taken in context to make an accurate diagnosis, which may lead to specific anatomic localization. Although the MRI does not show a structural lesion, it may be possible to infer the existence of such a lesion from clinical investigations. A focal neurological deficit offers presumptive evidence for such a lesion. Focal hypometabolism in a PET scan, lack of expected activation in a cortical region with fMRI, and DTI abnormalities all suggest the presence of a lesion responsible for epilepsy and can convey a good surgical prognosis (6–10). Detection of a focal abnormality in these tests can aid in planning the location of intracranial electrodes. Other tests, such as the scalp EEG and MEG, are often helpful in identifying areas that could be involved in seizure generation and therefore guide the intracranial investigation. In contrast, the authors have not found neuropsychological test results to be particularly useful in planning an intracranial investigation (and the literature contains little evidence to support the localizing value of these tests). People with refractory epilepsy typically have multifocal deficits, and when epilepsy begins in childhood, reorganization of various functions further muddies the waters.
As noted above, it is critical to synthesize data from different sources to develop a hypothesis to guide electrode implantation. Subdural electrodes can then be placed to record EEG from suspect neocortex and define the boundaries of the area to be excised. If buried cortex is implicated (e.g., hippocampus or the depth of a gyrus), then depth electrodes might be used in concert with subdural electrodes for optimal sampling of suspect brain areas. In an ideal circumstance, the noninvasively obtained information provides a strong indication of the source of seizures, and a fairly restricted area of brain needs intracranial EEG sampling. Sometimes, however, broad areas of neocortex are suspect in one or both hemispheres, e.g., both frontal lobes, and a second intracranial electrode study must be performed after the first intracranial EEG study narrows the focus to a particular region of the brain. This region can then be intensively studied and mapped to optimize outcome after surgery.
Types of electrode placement for different types of MRI-negative epilepsy
Frontal lobe epilepsies: The frontal lobes contain several discrete cortical areas which produce a variety of distinct types of seizures with different semiology. When clinical suspicion is directed to particular areas, a suitable strategy for placing intracranial electrodes can be suggested. One should always avoid focusing electrode placements in too narrow an area, since the clinical hypothesis might be incorrect. Sampling from adjacent areas or lobes often improves confidence in localization and helps prevents surprises.
1. Supplementary motor or cingulate seizures: medial frontal cortex can be studied with either subdural or depth electrodes. Subdural electrodes offer the advantage of placing many contacts in one or both hemispheres, and allow for mapping of the spatial extent of the seizure onset. Electrode strips or grids can be placed in interhemispheric cortex in an anterior to posterior direction and parasagittal electrodes can supplement these electrodes to define the lateral extent of resection. In this region, the same electrodes can be used to electrically stimulate the brain to define primary motor and sensory cortex, and somatosensory evoked potentials can be recorded to aid in defining these same areas. Stereotactically placed depth electrodes can also be placed in this region, but the amount of cortex sampled may be more limited with these electrodes.
2. Orbitofrontal seizures: orbitofrontal cortex can be sampled with subdural grids or strips. Placing grids has greater potential for morbidity because of the need to mobilize the frontal lobe during grid placement. Defining the anterior–posterior extent of an orbitofrontal epileptogenic zone can be challenging. The anterior perforated space is a natural boundary for any resection and investigation in this region. Rapid spread of orbitofrontal seizures to the amygdala and vice versa poses challenges, and simultaneous recording from amygdala with depth electrodes is usually advisable when an orbitofrontal focus is suspected.
3. Dorsolateral frontal lobe seizures: subdural grids are ideally suited for sampling from large areas of dorsolateral frontal lobe, and mapping of eloquent cortex with electrical stimulation and somatosensory evoked potentials.
4. Frontal pole seizures: subdural strips or grids can be used, with the former usually adequate to define the posterior margins of any resection.
Parietal lobe epilepsies: Smaller than the frontal lobes, both medial and dorsolateral parietal cortex can be studied with subdural electrodes. The parietal lobes are often included in intracranial surveys when the frontal lobes are studied with intracranial EEG.
1. Medial parietal seizures: the technique is identical to that employed for medial frontal seizures.
2. Dorsolateral parietal seizures: the technique is identical to that used for dorsolateral frontal lobe seizures.
Occipital lobe epilepsies: The occipital lobes have anatomic connections with both parietal and temporal lobes due to ontogeny, with superior occipital regions projecting preferentially to the parietal lobe, while the inferior portions (below calcarine sulcus) project to the temporal lobe. Hence, areas in these lobes should usually be evaluated when occipital seizures are studied.
1. Lateral occipital lobe seizures: the technique is the same as for lateral cortical foci in frontal and parietal lobes. Subdural grids or strips may be used.
2. Medial and basal occipital lobe seizures: interhemispheric and basal strips or grids can be used. Seizure onsets can be more easily mapped with grids, but surgeons infrequently excise striate cortex for epilepsy, so the intracranial EEG technique should not be overly aggressive if resection will not be done in that area.
1. Mesial temporal lobe seizures: Subdural electrodes can be used to record from parahippocampal gyrus, but amygdala and hippocampus are buried and subdural electrodes record at a distance from these structures. Hence, depth electrodes are better suited for intracranial EEG recording from mesial temporal structures in most circumstances (11).
2. Basal temporal lobe and temporal pole neocortical seizures: subdural electrode strips are ideally suited to record from these regions. While one could use a subdural grid, this is usually not necessary.
3. Posterior temporal lobe neocortical seizures: subdural grid electrodes are well suited to map the seizure onset zone and define areas critical for language (Wernicke’s area). Arrays of subdural strips can also be used, but intraoperative language mapping is more often required due to gaps in sampling from cortex with strip electrodes.
Pediatric considerations: Invasive monitoring is less often indicated in children than adults because of the higher prevalence of lesions in the MRI. When necessary, intracranial monitoring sessions tend to be briefer to enhance patient tolerance of the procedure. Subdural electrodes are the preferred type of electrodes for most pediatric studies. Mesial temporal epilepsy is uncommon in surgically treated young children, and this constitutes a major reason to use depth electrodes. Stereotactic EEG can be used, but there is insufficient published experience to compare it with subdural recording. Mapping of cortical function can be accomplished in children, but developmental considerations often limit the utility of this technique, and because of the inability of many children to fully cooperate with testing. Fewer electrodes are placed in children than adults because of small size of the skull, though this paradoxically permits more extensive sampling of cortex. Management of fluid and electrolyte balance is more complex, bridging veins more fragile, and anchoring of electrodes might be more challenging. Nonetheless, with a well-focused question, subdural grids and strips can be used with good results in small children (12).
Interpretation of subdural EEG
Several basic principles underlie interpretation of the intracranial EEG (Table 10.3).
Table 10.3 Intracranial EEG interpretation
One must carefully examine the interictal EEG. Underlying gliosis may be suspected when observing disruption of normal background rhythms, with loss of faster frequencies. Delta activity is generally not significant; a variety of temporary factors related to surgery can cause delta. The amplitude of intracranial EEG signals also is not important. Amplitude can be biased by the presence of blood beneath the electrode, and it depends upon the relationship between the recording electrode and the solid angle of dipole being recorded.
Interictal spikes can be meaningful and should be factored into surgical decisions. They are more widespread in the intracranial EEG than scalp EEG, often seen in both hemispheres and in multiple lobes. Interictal spike rates may not be higher in the region of the epileptogenic zone, and they must be considered more carefully (Figure 10.3). Sporadic spikes are usually not significant. Periodic, autonomous, repetitive spikes are perhaps more often associated with pathologic cortex. Gamma bursts lasting 100 milliseconds to several seconds also may be present, and usually are pathologic, though they are not always confined to the epileptogenic zone in our experience. When spikes are located in the area of an underlying disturbance of background rhythms, they carry greater significance as well. In certain conditions such as focal cortical dysplasia, intracranial spikes help delineate the extent of the dysplasia (13). One must establish the relationship between interictal spikes, the area of disrupted background activity, and the ictal onset zone. The surgical resection should ideally include areas with pathological interictal findings as well as the areas where seizures appear.
Figure 10.3 Interictal EEG showing sporadic spikes in both hemispheres. They are present in right and left hippocampal contacts (RAP, RMP, RPP, LAP, LMP, LPP), and right and left temporal lobe subdural contacts (RAT, LAT) (though not in the right temporal subdural electrode RST).
The ictal EEG contains the most desirable data, since it presumably is a good indicator of the epileptogenic zone – the real source of seizures. Figure 10.4 shows an example of a temporal lobe neocortical seizure recorded with subdural and depth electrodes. Neocortical seizure onset most often manifests with frequency beta (> 13 Hz) or gamma (> 30 Hz) activity, but occasionally slower frequency activity or an increased rate of interictal spiking may be the first sign of a seizure. The subdural EEG may show a well-localized, highly restricted area in which seizures first appear (Figure 10.5), or a broader area contained within a single lobe, as in Figure 10.4, or even multilobar onset. Highly focal gamma is probably the best indicator of the zone of seizure onset, but undue reliance should not be placed on this zone as the definitive marker for resection. As discussed below, only 50% of patients identified by intracranial EEG (meaning primarily ictal recording) become seizure-free after surgery. The ictal EEG is far from the optimal tool. Areas of early spread of ictal activity should be noted as well, since evidence suggests that excision of secondarily activated areas can be associated with improved outcome (12). In addition, the rate of propagation of seizures to the opposite hemisphere has been related to surgical outcome. Longer interhemispheric propagation times are associated with better outcome after temporal lobe (14) and extratemporal (15) resection. The EEG of seizures may be relatively stereotyped in any one individual, although nearly all patients have some degree of variability, however modest. In other patients, the EEG may display more than one EEG onset pattern, particularly when more than one area generates seizures. Patterns of spread can also vary, and termination patterns might differ somewhat from one seizure to the next as well.
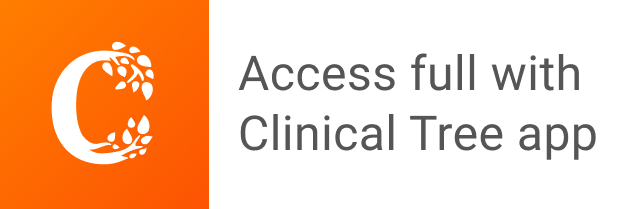