Chapter 11 Depth electrode and stereoelectroencephalography in MRI-negative epilepsy
MRI-Negative Epilepsy, ed. Elson L. So and Philippe Ryvlin. Published by Cambridge University Press. © Cambridge University Press 2015.
Introduction
Stereoelectroencephalograpy (stereo EEG or SEEG) primarily refers to the capacity of recording intracerebral EEG from various cortical targets, for which precise anatomical delineation and implantation requires a three-dimensional stereotaxic procedure. This approach appears well adapted to the investigation of cerebral networks distributed over several lobes or sublobar structures, including deeply located as well as more superficial cortical areas. Such investigations are particularly useful in patients with MRI-negative refractory focal epilepsy, whose main presurgical issues can be summarized as follows:
1. The lack of an identifiable epileptogenic lesion on MRI usually translates into uncertainties regarding the sublobar region or even the lobe generating seizures, justifying the need for intracerebral EEG (icEEG) investigations that enable sampling of all suspected regions.
2. MRI-occult type II focal cortical dysplasia (FCD) represents the most common pathology disclosed in successfully operated patients, and is often located in deeply located brain regions that are best sampled with depth electrodes.
3. The possibility of very large or multifocal epileptogenic zones, often associated with type I FCD, also needs to be considered, and further justifies icEEG targeting complex distributed networks.
While SEEG offers many advantages when investigating patients with MRI-negative refractory focal epilepsy, it also raises some issues: 1. SEEG does not represent a unified approach, but rather encompasses a variety of concepts and methodologies which makes it difficult to summarize its clinical effectiveness; 2. validation of the various forms of SEEG investigation remains scarce, and primarily based on the success rate of SEEG-guided epilepsy surgery in observational studies. This is even truer for MRI-negative patients, for which no randomized trial is available for the comparison between subdural grids and SEEG.
In this chapter, we will first present the historical and conceptual frameworks of SEEG, together with their evolution over time and variation between centers, the underlying surgical methods and associated complications, and the effectiveness of SEEG in patients with MRI-negative refractory focal epilepsy. Low- and high-frequency stimulations, as well as thermolesions will also be discussed.
Conceptual frameworks and disputed issues in SEEG
Historical perspective
The original SEEG method was developed in the late 1950s by Talairach and Bancaud at St Anne hospital in Paris (1, 2, 3), taking advantage of their progress in the field of brain stereotaxy (4). It should be stressed, however, that brain stereotaxy was also developed since the mid-1940s by Spiegel and Wycis who performed thalamic EEG recordings in patients with epilepsy (5, 6). In the very early stages of SEEG, intracerebral EEG was recorded acutely in the operating room, while brain targets were primarily guided by clinical and scalp EEG findings, long before CT scan and MRI were made available. The fact that SEEG was developed when brain targets could not be guided by neuroimaging, but rather by the electroclinical pattern demonstrated during scalp EEG recording of seizures, might account for its specific relevance for investigating MRI-negative patients (7).
The SEEG technique, as defined by Bancaud and Talairach, was selectively performed at St Anne hospital in Paris for decades, while a few other centers were perfoming more standardized depth electrode recordings focusing on the temporal lobes (8). During the last 25 years, SEEG has slowly disseminated to many French centers as well as to Italy (Milano) (9). More recently, a dramatic worldwide development of SEEG has been observed, triggered by the progress in frameless stereotaxy, and promoted by the international SEEG course. The SEEG method is thus entering a new era, more than 50 years after its invention (10, 11).
The anatomo-electro-clinical correlation theory
While SEEG terminology primarily refers to the stereotaxic implantation of intracerebral electrodes, the main conceptual framework underlying the SEEG method developed by Bancaud and Talairach lies in the so-called “anatomo-electro-clinical correlations,” whereby the ictal discharge is viewed as a spatiotemporal dynamic process, the comprehensive understanding of which promotes an accurate delineation of the epileptogenic zone (12–15). Neuroimaging and EEG findings accumulated during the last 50 years have largely confirmed and enriched the anatomo-electro-clinical correlation theory developed by SEEG founders. However, these data also stress that the spatiotemporal dynamics of epileptic seizure is more variable and complex than initially thought, to the point that a “comprehensive understanding” of the dynamics at the individual level is often elusive. At best, SEEG will sample about 1% of the total brain volume, hampering accurate extrapolation of seizure activity in all unexplored regions, regardless of the thoughtful strategy used to optimally choose the SEEG targets. This caveat is often neglected, promoted by the subjective impression that one can build a mental representation of the propagating epileptic discharge that seemingly fits with the recorded SEEG data. As for models of electrical or magnetic source imaging, where an infinite number of solutions can account for the same EEG or MEG data set, interpretation of SEEG findings might appear consistent with the recorded data and yet be inaccurate. This issue is aggravated by the very limited cortical sampling of SEEG and the lack of objective and appropriate mathematical models to display seizure propagation in clinical practice. Recent attempts to provide three-dimensional representation of an “epileptogenicity” index are thus of interest (16), but only partly address the above limitations.
Disputed issues regarding seizure propagation areas
While the quest for a better understanding of seizure propagation is justified by the fact that propagation patterns partly depend on, and thus can inform about, the seizure onset zone, the extent to which this should influence individual SEEG plan ought to be disputed. An illustrative example would be that of a patient with dialeptic seizures that would at times progress towards clonic twitches of the right arm during the latest stage of the attack. According to these observations, some SEEG centers would investigate the left central region on top of those suspected to generate seizures, while others would consider it useless to place an electrode in a brain region, the involvement of which during the late phase of the ictal discharge, but not during its early phase, can be fully ascertained based on ictal phenomenology alone.
Another disputed issue regarding the general concepts underlying SEEG is that of the significance of early or rapid seizure spread. Some authors consider that brain regions sustaining such early propagation need to be resected together with the seizure onset zone (17–20). Others would apply this statement only if a low-voltage, high-frequency discharge is observed in these regions. These diverging views solely rely on personal experience without any available validation study. Moreover, the empirical observation that extending the surgical resection to brain structures involved in early seizure spread might provide greater chance of postoperative seizure freedom could simply reflect the fact that the larger the corticectomy, the more likely it is to include the entire epileptogenic zone, regardless of the role of early propagation.
Overall, there is no consensus among SEEG centers on how to investigate and interpret regions of seizure spread, translating into different implantation strategies.
Current SEEG framework in MRI-negative patients
As discussed in the introduction, patients with MRI-negative refractory focal epilepsies raise specific issues that justify performing a SEEG investigation, but also determine its overall strategy. These issues derive from the uncertain etiology, number, extension, and localization(s) of the epileptogenic zone(s), together with the limited spatial sampling of SEEG. As a rule, a “fishing expedition,” i.e., a largely distributed implantation without any strong hypothesis, must be avoided, since the latter is very likely to either fail at detecting a seizure onset zone or provide an erroneous localization of the latter. Thus, SEEG should only be performed in patients where noninvasive data consistently point to a sublobar region (11, 14). In some patients, noninvasive data are consistent and compelling enough to obviate the consideration of an alternative hypothesis, in which case, SEEG will only be performed to define the extent of the epileptogenic zone. In other patients, uncertainties regarding the location of the epileptogenic zone lead to consideration of one primary hypothesis together with one or several less likely alternatives. While SEEG allows us to test several such hypotheses, it cannot offer optimal sampling of all tested regions. Indeed, the investigation of the most suspected epileptogenic region will typically require the implantation of five to ten electrodes within and around this region to precisely identify its borders (depending on its anatomical configuration). According to the maximum number of implanted electrodes (usually ≤ 15), it is difficult to use the same design for alternative seizure onset zones, where only one or two “sentinel” electrodes will be typically inserted (Figure 11.1).
Figure 11.1 Theoretical design of a SEEG investigation. Small circles (N = 14) represent intracerebral electrodes, with the blue corresponding to those implanted in the ictal onset zone, those in green placed in the surrounding propagation areas, and those in brown testing alternative seizure onset zones. The configuration of blue and green electrodes should allow for fine delineation of the borders of the ictal onset zone.
Two main clinical situations ought to be distinguished to further develop SEEG strategy in MRI-negative patients:
a. Mesial temporal or temporolimbic epilepsies, usually associated with very suggestive electroclinical and FDG-PET features, for which the main incentives for performing SEEG are: 1. excluding bitemporal, pseudotemporal, or temporal “plus” epilepsies (21, 22), all of which can mimic typical mesial temporal lobe epilepsy (mTLE) (23); and 2. exploring the possibility of a selective temporopolar (24), amygdala, or entorhinal (25, 26, seizure onset zone that will allow sparing part or all of the nonatrophic hippocampus in order to reduce the risk of postoperative memory decline, in particular when operating on the side dominant for language (27). According to these objectives, depth electrodes are required to selectively target the various mesial temporal structures of interest (see above), as well as the insula, orbitofrontal cortex, or mesial temporo-occipital or temporoparietal junction when judged necessary. Excellent seizure outcome has been reported in well-selected groups of MRI-negative mTLE with either end-folium sclerosis or negative as well as nonspecific pathology (28–33).
b. All other epileptogenic zones, often quoted as neocortical, for which the main hope and objective of SEEG is to disclose and delineate a MRI-occult FCD. Indeed, the great majority of patients with MRI-negative neocortical epilepsy who benefited from successful epilepsy surgery proved to suffer from such pathology, usually FCD type II (7, 14, 34, 35). Conversely, less than a handful of patients with normal pathology were reported to be cured from their epilepsy by extratemporal lobe surgery. Thus, in contrast with cryptogenic mTLE where presurgical investigations primarily aim at confirming the temporal origin of seizures, the work-up of MRI-negative extratemporal epilepsies should equally search for evidence of an underlying FCD as for their seizure localization. In this context, the design of the SEEG plan also needs to consider the spatial characteristics of MRI-occult type I and II FCD. The MRI-occult type II FCD will typically be small-sized and located at the bottom of a single sulcus, with some predilection for specific brain regions such as the superior frontal sulcus in the frontal lobe (7, 34–36). Conversely, MRI-occult type I FCD might be extensive, involving several gyri and sometimes several lobes, and also multifocal. Accordingly, SEEG strategy will differ as a function of the most likely underlying pathology.
All noninvasive investigations are useful to elaborate the most relevant hypothesis and related SEEG plan. Auras, when present, often have a high localizing value. Early ictal signs and scalp EEG changes also prove instrumental. When available, ictal SPECT and SISCOM bring additional useful information. Interictal EEG, MEG, and FDG-PET abnormalities are important not only for localizing the epileptogenic region but also to unravel its underlying pathology. In particular, FCD type II will often be characterized by intense spiking activity and marked glucose hypometabolism with clear-cut borders (35, 37). Coregistration of electric source imaging or MEG clusters, together with SISCOM or PET findings, helps in designing an optimal SEEG plan to precisely target the core and borders of suspected type II FCD (38). Past history, including age of onset of epilepsy, can also help inferring the underlying pathology (7, 34, 35, 39).
Figure 11.2 Typical placement of depth electrodes in the temporal lobe of patients with MRI-negative mTLE (other electrodes might be placed outside the temporal lobe according to patient’s characteristics). Electrode’s anatomical targets: J = temporal pole, D = entorhinal cortex and anterior portion of 3rd and 4th temporal gyri, L = posterior aspect of 3rd and 4th temporal gyri, A = amygdala and anterior portion of second temporal gyrus, B = anterior hippocampus and anterior portion of second temporal gyrus, C = posterior hippocampus and posterior portion of second temporal gyrus, T = anterior/mid portions of first temporal gyrus (reaching the anterior part of the long posterior gyrus of the insula), H = posterior portion of first temporal gyrus (reaching the posterior part of the long posterior gyrus of the insula).
Surgical aspects of SEEG methodology and their evolution over time
The surgical methods used to perform SEEG have evolved over time in parallel with its dissemination, according to three main stages. In the preMRI era, stereotaxic ventriculography and angiography were performed and superimposed to delineate the AC–PC line, define atlas-guided anatomical targets, and ensure maximal vascular safety of orthogonally implanted electrodes.
Figure 11.3 Advantage of SEEG in investigating MRI-occult type II focal cortical dysplasia. The MRI on the left illustrates the typical location of a type II focal cortical dysplasia at the bottom of sulcus. The image on the right shows the recording from an electrode targeting a MRI-occult FCD in the anterior insula. High-amplitude spikes are observed over the bipole P’1–P’2 (i.e., insular leads), but not detectable 1 cm more laterally within the frontal cortex.
When MRI became available, it rapidly replaced ventriculography and atlas-guided implantation, by providing direct targeting of the brain structures to be investigated. However, most SEEG centers have continued to use orthogonal implantations coupled with stereotaxic angiographies with X-ray sources located 5 m away from the patient’s head, to eliminate the linear enlargement due to X-ray divergence, offer optimal stereotaxic registration, and minimize the risk of vascular injury. More recently, digitization of angiography data has allowed the replacement of the cumbersome 5 m-distant X-ray sources by flat-panel X-ray detectors. The safety of this method, detailed below, has enabled the expansion of the type and number of brain regions targeted by SEEG, leading to the investigation of the insular cortex and implantation of up to 15 electrodes in the same individual. However, this approach remains limited by the orthogonal trajectory of all implanted electrodes.
The third era is that of robot-assisted stereotaxy allowing for double-oblique trajectories. This method, initiated in Grenoble (France) (40), and further developed in Milano (Italy) and Marseille (France), is now rapidly expanding with the use of MR angiography as a substitute for conventional angiograms.
Current methodology for orthogonal implantation using the Talairach’s grid
Under general anesthesia, the patient’s head is fixed in the stereotaxic frame (typically the Talairach’s frame, but the Leksell’s frame can be used as an alternative provided it is adaptated to the Talairach’s metallic grid) using transcutaneous metallic pins. Stereotaxic digitalized angiography is then performed using flat-panel X-ray detectors attached to the operating table (typically two, lateral and anteroposterior). Angiography should involve the common carotid artery, in order to display both intracerebral and anterior meningeal arteries, and provide arterial and venous views. The previously acquired frameless MRI is then coregistered to the digitized angiogram, using anatomical landmarks of the corpus callosum provided by the anterior cerebral artery and vein of Galen. Each MRI-based brain target is then adjusted to allow an avascular trajectory of the corresponding electrode, and transformed into coordinates of the Talairach’s grid. A metallic landmark is then inserted in the Talairach’s grid at each of the calculated coordinates, in order to check by means of a standard X-ray view, their appropriate placement with respect to the original anatomical targets and vascular constraints. Percutaneous trephination is then performed through the selected holes of the Talairach’s grid. It should not include the dura, which will then be carefully coagulated. A hollow peg is inserted in each trephination hole, and its position checked by X-ray views. The distance between the internal extremity of the peg and the desired depth of the tip of the electrode is then calculated, given a 5 mm safety distance to any cisterna. Electrodes are then inserted according to these calculations, with a fine adjustment of the penetration length being performed on the basis of X-ray views obtained at the end of the procedure. Electrodes are then fixed to the peg by means of a plastic cap. The precise anatomical location of each recording lead is later provided by MRI scan (27).
Current methodology for robot-assisted oblique implantation
Oblique trajectories offer several advantages over classic orthogonal electrodes, which include targeting regions not attainable through the Talairach grid (e.g., rectus gyrus, occipital pole), increased sampling of brain structures, the long axis of which is sagitally oriented (insula, cingulate gyrus), and optimized placement of the recording leads within the gray matter (41). However, it also has some drawbacks, including more complex 3D representation for clinical interpretation of data. Furthermore, when investigating cortical networks primarily connected through coronally disposed fibers, orthogonal electrodes allow a more straightforward understanding of such network activity (e.g., the insulo-opercular complex or mesiolateral parietal network, etc). Some oblique trajectories also allow for implanting depth electrodes without angiography control.
Several methods of robot-guided SEEG have been developed, the technical characteristics of which will not be detailed in this chapter. In any case, electrode trajectories are determined on the patient’s MRI through the robot-dedicated software. This procedure requires fiducials to be inserted in the patient’s skull, either at the time of MRI, or during a perioperative additional scan, which is then merged with MRI. Conventional digitized angiography or MR angiography can also be incorporated into the robot’s software to minimize the risk of vascular insult. Similarly, other coregistered information can be used for refining the implantation strategy, such as diffusion tensor imaging and tractography, functional MRI, nuclear medicine data (SPECT or PET), or electrical/magnetic source imaging. Combination with the Talairach’s frame and grid is possible, and can allow mixed procedures with both orthogonal and oblique electrodes. Trephination and procedures to insert and secure the electrodes are not different from those described for orthogonal implantations.
Cardinale and collaborators have evaluated the accuracy of traditional versus modern robot-assisted procedures on 118 of their 500 SEEG procedures (42). The traditional method was associated with a median entry point localization error of 1.43 mm (interquartile range, 0.91–2.21 mm), and a median target point localization error of 2.69 mm (interquartile range, 1.89–3.67 mm), versus a median entry point localization error of 0.78 mm (interquartile range, 0.49–1.08 mm) and a median target point localization error of 1.77 mm (interquartile range, 1.25–2.51 mm) for the current robot-guided procedure (42).
SEEG complications
SEEG is usually considered a safer and better-tolerated investigation than subdural grids. However, no randomized comparison of the two methods is available, and only a few systematic evaluations of SEEG complications have been reported.
The Italian group of Milano have reported the largest series of 500 consecutive SEEG with a total of 6496 implanted electrodes (42). Twelve major complications were encountered (2.4%), including one death in a 3-year-old child whose autopsy showed massive cerebral edema without intracranial hemorrhages, suggesting an impairment of the hydroelectrolytic balance of unclear origin. Five intracranial hemorrhages were also observed (1%), including one extradural during a preimplantation procedure, one subdural, and three intraparenchymal, two of which resulted in permanent motor deficit. Two patients developed intracranial infections. One obstructive hydrocephalus, caused by a small clot in the aqueduct of Sylvius, required temporary external ventricular drain, while one broken retained electrode had to be surgically extracted. Interestingly, all these complications occurred during the first 215 procedures (18).
The second largest series was reported by the MNI group (Montreal, Canada), and included 224 implantations with 3022 electrodes (43). Ten major complications were reported (4.5%), including three hematomas (1.3%), three brain abscesses, one meningitis, and one hypointense lesion found 1 week after electrode explantation. Scalp cellulitis was observed in an additonal four patients, while two patients developed hemiparesis during preoperative angiography in the early 1980s (43).
Our group reported SEEG complication rates in our first 100 patients in whom 1118 electrodes were implanted. There were three major complications (3%), including one intracerebral hematoma (1%) which resulted in death, and two electrode breakages (27). The death was indirectly related to the procedure since it occurred several days after the electrodes were removed. The patient had developed brachial thrombophlebitis during the late phase of SEEG recordings and was transferred to a cardiovascular surgery department with a view to proceeding to surgical treatment of the venous clot. Eventually, vascular surgeons decided to perform to intravenous thrombolysis, despite the contraindication of a recent brain surgery, which resulted in the fatal hematoma. We have now updated our complication rate to 460 SEEG procedures. In addition to the three above complications, four hematomas were observed, including three asymptomatic subdural or extradural hematomas, and one intracerebral that resulted in permanent language deficit. Another four electrode breakages occurred, without neurological consequences, and two rapidly controlled cases of meningitis. In total, SEEG-induced hematoma was observed in 0.9% of our patients, with permanent deficit in 0.2%, figures that are very comparable to those reported by the Milano group (1% hematoma, including 0.4% with permanent deficit).
In a more recent series of 100 patients in whom 1310 electrodes were implanted, the rate of hemorrhagic complications was 3% without permanent deficit (10).
A fatal hematoma was also recently reported in a 10-year-old boy, 8 days after electrode implantation, suggesting the rupture of a SEEG-induced growing pseudoaneurysm or the tearing of a neighboring vessel by an electrode (44).
Overall, major complications occur in about 3% of SEEG procedures, including 1% to 3% of intracranial hematoma, some of which will result in permanent deficit or death (≤ 0.6%).
SEEG recording and stimulation procedures
The SEEG technique offers the possibility of performing various types of electrical stimulation in order to test the epileptogenicity and functionality of implanted brain regions (45, 46).
Traditionally, high-frequency 50 Hz stimulations of 0.3 msec biphasic square pulses are delivered using trains of 5 seconds maximal duration, and 3 mA maximal intensity (47–49). Such stimulation, when applied to brain regions involved in the epileptic network, may generate: 1. ictal symptoms with or without associated EEG postdischarge (50–52), 2. subclinical postdischarge, and 3. full-blown seizure. In eloquent regions, high-frequency stimulation can generate positive symptom or deficit reflecting the brain function at stake (e.g., auditory hallucination, aphasia) (53–56). Functional mapping of language or sensorimotor areas is however less informative than that performed using subdural grids, due to the less extensive coverage of those regions by SEEG.
Low-frequency 1 Hz stimulation, using 1 msec duration pulse, up to 30 second duration and 5 mA intensity, appears more appropriate for testing the epileptogenicity or functionality of brain regions that are highly sensitive to electrical stimulation, such as the mesial temporal cortex or the central region (57, 58). In particular, hippocampal discharges elicited by low-frequency stimulation are thought to more reliably indicate an underlying epileptogenicity than those triggered by high-frequency stimulation (57, 59). Epileptogenic zones located outside the mesial temporal regions are more rarely activated by low-frequency stimulation (59). In the central region, low-frequency stimulation will elicit brief repetitive motor signs or sensory symptoms with lower risk of uncomfortable or painful sensations than those which might be triggered by high-frequency stimulation.
Very low-frequency stimulations at 0.2 Hz, also referred to as single pulse electrical stimulation, do not usually trigger epileptic discharges nor ictal signs or symptoms. However, they allow capturing of evoked potentials in other recorded regions. Some of these potentials are physiological, especially those occurring during the first 100 msec poststimulus, providing information on functional connectivity (60–63). Others, usually more delayed and polyphasic, are thought to reflect the epileptogenicity of either the stimulated or the recorded brain region (64, 65).
Effectiveness of SEEG in patients with MRI-negative refractory focal epilepsy
There are only a few series reporting SEEG findings in populations of 20 or more MRI-negative patients, most of which will also include patients with MRI abnormality (10, 14, 18, 35, 38) (Table 11.1). Most other series either were published before the MRI era or did not provide any details regarding MRI-negative patients, reported less than 20 patients with a normal MRI, or selected patients successfully operated to ensure the localization of the epileptogenic zone and its association with other clinical parameters.
Table 11.1 Effectiveness of SEEG in MRI-negative refractory focal epilepsy
The 2005 series from Milano is the first and largest study providing relevant information on SEEG findings and its impact on epilepsy surgery in a large population of MRI-negative patients (18). They reported 211 patients, 77 of whom (36%) had a normal MRI; SEEG delineated the epileptogenic zone in 204 patients (97%). Epilepsy surgery was proposed in 185 patients (88%), performed in 174 (82%), including 165 with a postoperative follow-up of ≥ 1 year, and 46 (28%) with a normal MRI. In this latter population, rates of complete seizure freedom and class I of Engel outcome (free of disabling seizures) were 27% and 38%, respectively, compared with 52% and 62% for MRI-positive patients. Pathology proved abnormal in 40 of the 46 (87%) MRI-negative operated patients.
The Marseille group reported a series of 100 consecutive patients who underwent SEEG, including 43 whose MRI was normal (14, 66). The epileptogenic zone could be delineated in 96%, while surgery was offered in 84%, with no difference in operability between patients with and without MRI abnormality. Complete seizure freedom was achieved in 53% of the 60 operated patients with a postoperative follow-up ≥ 12 months, including 55% of the 20 patients whose MRI was normal, and 53% of those showing MRI abnormality. Pathology in MRI-negative patients included focal cortical dysplasia (n = 7/9 seizure-free), gliosis (n = 4/7 seizure-free), hippocampal sclerosis (n = 1/2 seizure-free), and lack of available data in two (none seizure-free).
The Cleveland Clinic recently reported a similar series of 100 consecutive patients who underwent SEEG, including 61 whose MRI was either normal or showed extensive bilateral abnormalities, without details about each subgroup, however (10). Localization of the epileptogenic zone(s) could be achieved in 96% of cases, with 16% showing multifocal or bilateral foci which contraindicated surgery. These figures are remarkably similar to those reported above in the Marseille series. A total of 75 patients had surgery, 53 of whom had a postoperative follow-up ≥ 12 months. In this latter population, 28 patients (53%) had a normal MRI, with a 57% seizure freedom rate, versus 68% in those with an abnormal MRI. Pathology disclosed type I or II focal cortical dysplasia in most patients, and proved normal in seven (25%), only one of whom was seizure-free.
The St Anne hospital reported a series of 62 histologically proven type II FCD, 37 (60%) of which underwent SEEG prior to surgery, including 21 of the 25 MRI-negative (84%), and 16 of the 37 MRI-positive patients (43%, all before 2006) (35). The overall surgical outcome was 68% with complete seizure freedom (n = 42/62), and 92% with class I of Engel outcome (n = 57/62). Nonsignificantly greater seizure-free rate was observed in MRI-positive (75%) as compared to MRI-negative patients (56%), whereas the proportion of class I of Engel outcome was comparable in both groups (94% and 88% in MRI-positive and negative patients, respectively). The outcome data in the subgroup of patients who underwent SEEG were not provided.
Our group recently reported a series of 21 MRI-negative patients who underwent SEEG and MEG (38). A seizure onset zone was defined in all patients, but they were thought to be well localized in only 65%. Eleven patients had surgery, six of whom achieved a class I of Engel (55%). Pathology disclosed FCD in three patients, a mild form of malformation of cortical development not otherwise specified in another two, and proved normal in six patients.
Overall, less than 250 MRI-negative patients have been reported in large SEEG series; SEEG appears to enable a definite conclusion regarding operability in most patients (96%), about 80% of whom are subsequently operated. Complete seizure freedom rate in this challenging population varies between 27% and 56%, with most series providing figures > 50%. Class I of Engel outcome, when available, is substantially higher. The presence of an underlying FCD, demonstrated at pathology, is associated with greater chance of seizure freedom, whereas normal pathology is consistently associated with surgical failure.
SEEG-guided radiofrequency thermocoagulation
One specific advantage of SEEG over subdural grids, is the possibility to perform radiofrequency thermocoagulation (RFTC) lesions once the recording procedure is over, a technique developed by our group (67, 68). The RFTC is performed without anesthesia by using a radiofrequency generator connected to the electrode contacts. Lesions are produced between two contiguous leads from the same electrode, using a 50 V, 120 mA current, known to increase the local temperature up to 78–82°C within a few seconds. This leads to a spherical brain tissue lesion of about 5 mm in diameter, centered around the heated bipole, in 10–30 seconds. The SEEG recording is performed immediately before and after the procedure to check its impact on the interictal epileptiform discharges. The choice of SEEG targets and their number depend on the delineation of the epileptogenic zone. These targets are previously evaluated using bipolar electrical stimulation in order to avoid performing RFTC at eloquent cortical areas.
The RFTC can be used for various purposes. In difficult-to-operate patients with small FCD located in an eloquent area or in patients with periventricular heterotopic nodules, RFTC may allow the thermocoagulation of most of the epileptogenic zone to fully control seizures (69). In most patients, however, RFTC is used to further evaluate the extent of the epileptogenic zone. Specifically, a significant and prolonged reduction in seizure frequency is thought to reflect a rather limited epileptogenic zone around the thermocoagulated leads. Nine MRI-negative patients who underwent RFTC were reported by our group (70). Three (33%) had a reduction of seizure frequency greater than 50%, including two in whom the rate was 95%. Two of these patients later underwent surgery that consisted of a tailored resection of the amygdala and temporal pole not including the hippocampus. Both became seizure-free (class I of Engel). Among the six other patients where RFTC failed to decrease seizure frequency, four have been operated on, all of whom were seizure-free postoperatively (70).
Illustrative case: This is an ambidextrous 10-year-old boy, born in 1999, without any adverse past history and a normal cognitive development. Seizures started at the age of 7 and proved to be drug-resistant from the onset. Most seizures occurred at night and did not leave any memory of it in the patient. Conversely, daytime seizures started with paresthesia of the right side of the tongue, rapidly followed by a sensation of jaw locking and speech arrest. During this short-duration aura, the patient could not warn his family. Video recording of seizures showed that the patient rapidly developed an asymmetrical motor behavior, characterized by posturing of the right hemibody with the arm bent upward, while the trunk and left hemibody demonstrated hyperkinetic movements. Seizures were brief, lasting about 30 seconds without postictal confusion. There was no history of secondary generalization of the seizures. Overall, ictal phenomenology suggested a left-sided onset (right-sided paresthesias and posturing), rapidly involving the primary or secondary somatosensory cortex of the tongue and premotor/motor cortex of the jaw, but without other perisylvian sign (no hypersalivation, hemifacial tonic or clonic movement or paresis, mastication, laryngeal spasm, gustatory or olfactory symptom, vertigo, or auditory hallucination).
Interictal EEG consistently demonstrated left midtemporal spikes, more frequent during sleep (Figure 11.4); MEG demonstrated a spike cluster located in the inferior bank of the left perisylvian region. Using SAM analysis (synthetic aperture magnetometry), dipole modeling localized the source of the spikes within the left insula (Figure 11.5). Scalp EEG ictal onset was left-sided but without clear focalization at onset. The FDG-PET showed a clear-cut hypometabolism encompassing most of the left perisylvian region, with a maximal abnormality delineated by SPM analysis (statistical parametric mapping) in the left opercular region (Figure 11.6).
Figure 11.4 Interictal scalp EEG recording. Scalp EEG (referential montage) demonstrates spikes and polyspikes predominating over T3.
Figure 11.5. Magnetoencephalography. Upper row illustrates SAM (synthetic apperture magnetometry) analysis, with a spike cluster located in the inferior bank of the left perisylvian region. Lower row illustrates dipole modeling, showing a source of spikes centered within the left insula.
Figure 11.6 PET findings. The FDG-PET shows left perisylvian hypometabolism (white arrow), with a corresponding significant SPM cluster coregistered on MRI (green spot).
The SEEG plan was designed according to all the above data, with the primary hypothesis that the seizure onset zone was located within the midposterior and superior aspect of the left perisylvian region (i.e., posterior superior insula or frontoparietal operculum). However, we considered the alternative hypothesis of a temporal lobe onset according to the scalp EEG and MEG SAM findings. As illustrated in Figure 11.7, each main gyri of the suprasylvian opercular region were sampled with SEEG (P, Q, N, G), with the view that Q and N were the most likely candidates, and that P and G would provide appropriate anterior and posterior borders of the seizure onset zone. These four electrodes also investigated the insula, as well as electrodes T, U, and H which sampled its inferior aspect plus the temporal operculum. The less likely possibility of a temporolimbic onset was assessed by sampling the temporal pole (J), the amygdala (A), and the hippocampus (B, E). An orbitofrontal lead (F) was also inserted.
Figure 11.7 Strategy of SEEG implantation. Image on the left shows a sagittal MRI slice, cutting through the left perisylvian region, upon which all relevant imaging findings are superimposed: blue corresponds to visually detected hypometabolism covering the superior and inferior banks of the Sylvian fissure; green corresponds to the cluster provided by SPM analysis of FDG-PET data (contrasted with a database of 50 controls); yellow delineates the MEG focus provided by SAM analysis; red illustrates the cortical region activated during language fMRI. Black dots correspond to implanted electrodes whose targets were the following: A: amygdala and anterior middle temporal gyrus, B: anterior hippocampus and middle temporal gyrus, E: posterior hippocampus and middle temporal gyrus, F: orbitofrontal gyrus, G: posterior cingulate and supramarginalis gyrus, H: posterior inferior insula and superior temporal gyrus, N: posterior superior insula and parietal operculum, P: anterior superior insula and inferior frontal gyrus, Q: mid-superior insula and frontal operculum, T and U: anterior inferior insula and superior temporal gyrus.
The SEEG recordings demonstrated very focal interictal paroxysms and ictal onset within the posterior aspect of the frontal operculum (Q lateral), which propagated to the parietal operculum (N) (Figure 11.8). Seizures could be triggered by stimulating Q lateral, while the stimulation of F (Broca’s area) resulted in speech arrest.
Figure 11.8 SEEG findings. A very restricted area of almost permanent high-amplitude spikes is observed over the lateral leads of electrode Q 6–7, corresponding to the frontal operculum, with some slight propagations to the adjacent electrode N 6–7 (parietal operculum). Seizure onset was observed over the same leads. All leads are displayed with the same gain.
The RFTC was performed selectively on Q6–Q7 and Q7–Q8, showing an immediate disappearance of spikes, not only on all leads at electrode Q, but also on those at electrode N, suggesting that the spikes at the latter were propagated from Q (Figure 11.9). Thereafter, the patient was seizure-free for 3 months, before seizures relapsed. An awake corticectomy was performed, with a view to resecting the two small opercular gyri targeted by electrodes Q and N (Figure 11.10). Pathology demonstrated a type II FCD, and the patient has been seizure-free for 4 years.
Figure 11.9 Impact of thermolesion. Thermolesions were performed over q’6–7 and q’7–8 bipoles. High-amplitude spikes that were recorded over q’ and n’ leads immediately before the thermolesion, disappeared immediately after.
Figure 11.10 Surgery. A selective resection of the frontal operculum was undertaken, resulting in long-term seizure freedom (class IA of Engel) with no neurological deficit.
Conclusion and perspectives on SEEG
The SEEG technique has expanded very significantly over the last 5 years, in parallel with the increase in the number of patients with MRI-negative refractory focal epilepsy referred to surgery. Whether this association is coincidental or partly reflects the relevance of SEEG for investigating MRI-negative patients, is uncertain. In any case, the overall rates of informative investigation, surgical indication, and excellent seizure outcome support the use of SEEG in selected cases, in as much as its rate of major complications with either permanent deficit or death is reasonably low (≤ 0.6%). Finally, the development of frameless MRI-based and robot-assisted SEEG is facilitating the access to SEEG in new centers.
The comparative effectiveness of SEEG and subdural grids also remains a matter of debate, given the lack of controlled study addressing this issue. The dramatic shift from grids to SEEG in major epilepsy surgery centers with a huge experience in the former type of investigation, such as the Cleveland Clinic, suggests that SEEG might offer additional chances to accurately localize and remove the epileptogenic zone. In a series of 70 frontal lobe surgeries performed between 1995 and 2003 at the Cleveland Clinic, including 19 patients with a normal MRI, only 16% were seizure-free (71). Among the subset of 12 patients with an MRI-occult cortical dysplasia, only two patients were seizure-free at 1 year (16%), and only one at 2 years (8%). This contrasts with the 57% seizure-free rate reported by the same group in 28 MRI-negative patients investigated with SEEG (10), as well as with the 78% to 88% class I of Engel outcome reported by the Marseille’s and St Anne’s groups in a total of 30 patients with MRI-occult focal cortical dysplasia (14, 35).
The SEEG suffers a number of limitations, however, some of which could be tackled in the future. One important issue is the lack of guidelines for optimal indication, SEEG design (i.e., number and location of electrodes), and methods of implantation, with significant heterogeneity of practice between centers. Harmonization should be promoted, in as much as technological advances in the field progress at an increasingly rapid pace. Indications for SEEG may deserve a consensus statement. The great majority of successfully operated MRI-negative patients prove to suffer FCD at pathology, and most often FCD type II. The latter are usually associated with suggestive features, in terms of history (age of onset of epilepsy and drug resistance, seizure frequency and stereotypy over time), interictal FDG-PET and EEG/MEG findings. It may be debated whether patients with extratemporal seizures but no features supporting the hypothesis of an MRI-occult FCD should undergo SEEG. This issue is different in cryptogenic TLE where seizure freedom can be obtained despite normal pathology. However, whether performing SEEG with a view to sparing a normal-volume hippocampus is associated with clinically relevant benefit remains to be demonstrated. The same is true for the predictive value of thermolesions. Overall, many issues deserve to be addressed in the field of SEEG in the hope of increasing the yield of this investigation in MRI-negative refractory focal epilepsy.
References
















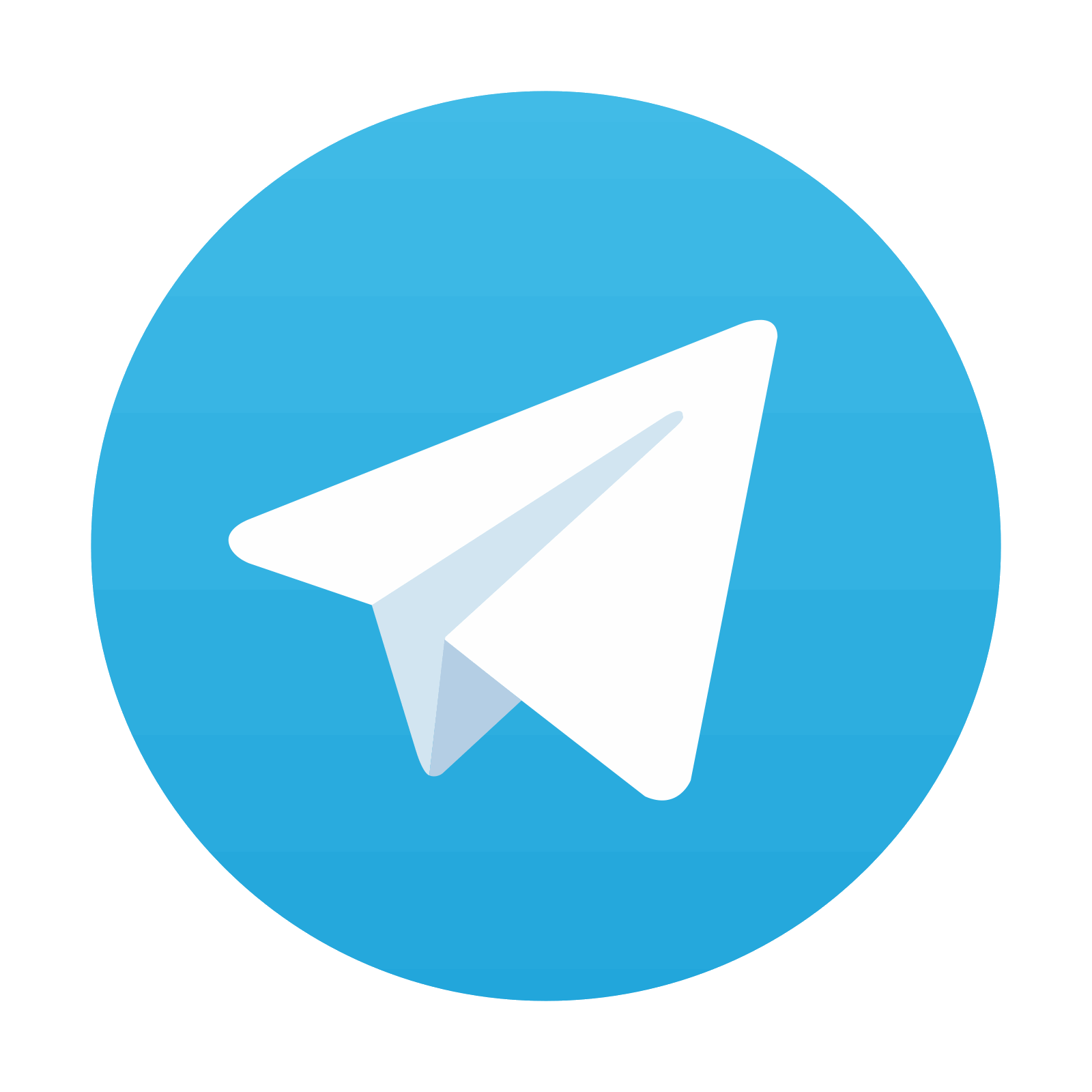
Stay updated, free articles. Join our Telegram channel
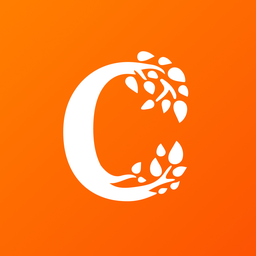
Full access? Get Clinical Tree
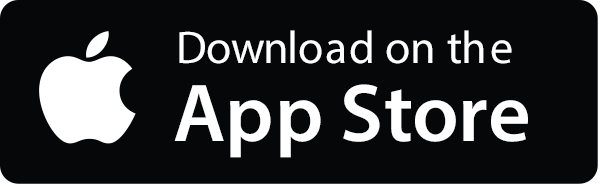
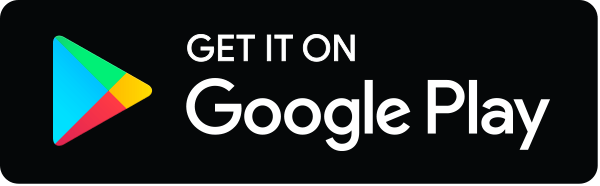
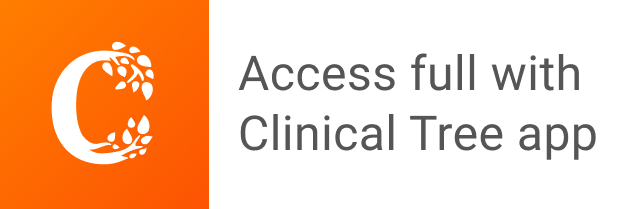