Abstract
Humans depend on dietary proteins as a source of amino acids; they are the metabolic basis of all functional and structural proteins in the body. Some amino acids – termed essential – cannot be synthesized by the human body, such L-isoleucine and L-phenylalanine. Renal conservation of amino acids is extremely effective, with clearance values mostly below 1%. Stool nitrogen losses are about 1 g per day and are mostly of bacterial origin. In contrast to glucose and fatty acids, amino acids taken in excess of requirement cannot be stored but their carbon backbones are used for energy production.
Overview
Humans depend on dietary proteins as a source of amino acids; they are the metabolic basis of all functional and structural proteins in the body. Some amino acids – termed essential – cannot be synthesized by the human body, such as L-isoleucine and L-phenylalanine. Renal conservation of amino acids is extremely effective, with clearance values mostly below 1%. Stool nitrogen losses are about 1 g per day and are mostly of bacterial origin. In contrast to glucose and fatty acids, amino acids taken in excess of requirement cannot be stored but their carbon backbones are used for energy production.
Amino acid disorders, organic acidurias, and urea cycle disorders are rare inherited disorders of protein metabolism caused by deficiencies of single enzymes, cofactor metabolism, or transporters involved in the oxidative breakdown of amino acids. Biochemically, they are characterized by the accumulation of metabolites upstream of the defective enzyme (i.e. amino acids, organic acids, and/or ammonium), causing intoxication. Enzyme deficiencies in the proximal part of amino acid catabolism result in the accumulation of precursor amino acids such as L-phenylalanine or branched-chain amino acids and thus are called amino acid disorders (AADs). Amino acids can be specifically detected by the ninhydrin reaction, which became available in the late 1940s, explaining why AADs belong to the earliest identified inborn errors of metabolism (IEMs). In contrast, the definitive breakdown of many amino acids occurs mostly within the mitochondria through degradation of coenzyme A (CoA)-activated carbonic acids, so-called acyl-CoA compounds. After hydroxylation of the CoA group, so-called organic acids (i.e. water-soluble mono-, di-, or tricarboxylic acids) are released. The pathological accumulation of characteristic organic acids, but not of precursor amino acids, in body fluids is the biochemical hallmark of organic acidurias (OADs), a disease group caused by an inherited dysfunction of the distal part of amino acid degradation. OADs became detectable after the introduction of gas chromatography, particularly gas chromatography/mass spectrometry (GC/MS), in the 1960s. Thus, the historic terminology of AADs and OADs is not based on pathophysiological differences but simply reflects the development of analytical approaches. It is noteworthy that GC/MS analysis has also identified IEMs of many pathways beyond amino acid degradation, for example of cholesterol metabolism, fatty acid oxidation, carbohydrate, or mitochondrial energy metabolism.
The breakdown of amino acids results in the release of neurotoxic ammonium that is detoxified by the urea cycle. The latter takes place in periportal hepatocytes and is composed of five catalytic enzymes, two associated enzymes producing a cofactor (N-acetylglutamate) and a substrate (bicarbonate) for the formation of carbamylphosphate, and two transport proteins. The efficacy of hepatic ammonium detoxification is enhanced through the action of L-glutamine synthetase in perivenous hepatocytes, resulting in the reversible fixation of excess ammonium to L-glutamate. In the brain, enhanced L-glutamine synthesis, mostly in astrocytes, is the only way to detoxify ammonium. The biochemical hallmark of most urea cycle disorders (UCDs) is thus hyperglutaminergic hyperammonemia.
Clinically, individuals with an inherited AAD, OAD, or UCD are usually born at term after an uneventful pregnancy and delivery, and are initially asymptomatic. The onset of the first symptoms is variable, ranging from metabolic decompensation within the first days of life to the late onset of symptoms during adulthood. If the diagnosis is delayed or missed, irreversible organ damage or early death may follow [1]. In childhood, metabolic decompensations are triggered by an excess intake of protein and, most importantly, the breakdown of body protein during episodes of catabolism. The disease spectrum is broad but follows a distinct pattern in specific disorders. Despite considerable clinical variability, the central nervous system is most often affected in AADs, OADs, and UCDs [2]. Recent pathogenic concepts are based on the observation that some pathological metabolites impair key intracellular functions, such as energy metabolism, and thus may become toxic at increased concentrations, causing irreversible damage or reversible dysfunction. Other metabolites interfere with neurotransmitter pathways or interact with neurotransmitter receptors. This might explain the specific vulnerability of the brain [3].
Major therapeutic strategies aim to restrict the intake of precursor amino acids (low protein or calculated diets specifically restricting precursor amino acids), stimulate residual enzyme activity (cofactor treatment), facilitate urinary excretion (hydration, forced diuresis), enhance physiological pathways of detoxification (e.g. formation of non-toxic acylcarnitines), or open alternative routes (e.g. nitrogen scavengers, extracorporeal detoxification) [4–7]. Finally, solid organ transplantation (liver, kidney) increases the residual activity of the defective enzyme and thus attenuates or even rescues the biochemical phenotype and improves the outcome [8]. However, since irreversible organ dysfunction is already found in newborns or infants, therapies are more effective if they can be started in affected individuals while asymptomatic. Based on the seminal work of Robert Guthrie and Horst Bickel, newborn screening programs and effective therapies for many intoxication-type IEMs have been successfully developed, highlighting that such preventive programs can be disease-changing [9].
This chapter focuses on AADs, UCDs, and OADs and discusses movement disorders in these groups of disorders (Table 12.1).
Table 12.1 Examples of AADs, OADs, and UCDs presenting with movement disorders
Disease | Inheritance* | Gene (location) | Enzyme(Name, EC or TC number) | Estimated prevalence (X per 100,000) | MIM number |
---|---|---|---|---|---|
Phenylketonuria (PKU) | AR | PAH (12q23.2) | L-phenylalanine hydroxylase (EC 1.14.16.1) | 10 | #261600 |
Methylmalonic aciduria (MMA, mutase-deficient type) | AR | MMUT (6p12.3) | Methylmalonyl-CoA mutase (EC 5.4.99.2) | 1 | #251000 |
Glutaric aciduria type 1 (GA-1) | AR | GCDH (19p13.13) | Glutaryl-CoA dehydrogenase (EC 1.3.8.6) | 1 | #231670 |
L-2-Hydroxyglutaric aciduria (L2HGA) | AR | L2HGDH (14q21.3) | L-2-hydroxyglutarate dehydrogenase (EC 1.1.99.2) | <1 | #236792 |
HHH syndrome | AR | SLC25A15 (13q14.11) | Mitochondrial L-ornithine transporter 1 (TC 2.A.29.19.2) | <0.1 | #238970 |
* AR, autosomal-recessive.
The clinical spectrum of major AADs, OADs, and UCDs is discussed in detail in this chapter and is summarized in Table 12.2.
Table 12.2 Clinical spectrum of individuals with AADs, OADs, and UCDs
Disease | Neurological manifestations | Other manifestations |
---|---|---|
PKU |
|
|
MMA (mutase-deficient type) |
|
|
GA-1 |
| Kidney: Chronic kidney disease (adults), inconsistent |
L2HGA |
|
|
HHH syndrome |
|
|
Amino Acid Disorders
Phenylketonuria
Phenylketonuria (PKU), first described in 1934, is found with an average frequency of about 1 in 10,000 newborns with large national and ethnic variability. It is caused by bi-allelic mutations in the PAH gene (gene locus: 12q23.2) encoding for the enzyme L-phenylalanine hydroxylase, which is expressed in liver and kidney and catalyzes the conversion of L-phenylalanine to L-tyrosine and requires tetrahydrobiopterin (BH4), iron, and molecular oxygen as cofactors. The metabolic consequences of PAH deficiency are increased plasma and cerebrospinal fluid (CSF) concentrations of L-phenylalanine and decreased L-tyrosine. Furthermore, phenylacetate, giving the urine a mousy odor, and other “phenylketones” accumulate [10]. Eighty years after its description, the molecular mechanisms of PKU are still not completely understood. L-phenylalanine competes with the transport of large neutral amino acids across the blood–brain barrier using L-type amino acid transporter 1. Since L-phenylalanine has the highest affinity to this transporter, increased plasma L-phenylalanine concentrations dramatically limit the transport of large neutral amino acids (LNAAs) to the brain, resulting in an overall reduced cerebral biosynthesis of proteins and neurotransmitters such as dopamine and serotonin (the so-called LNAA hypothesis) [11]. L-phenylalanine also exerts direct effects on glutamatergic signaling since it competes with L-glycine and L-glutamate at their binding sites in N-methyl-D-aspartate (NMDA) and alpha-amino-3-hydroxy-5-methyl-4-isoxazolepropionic acid (AMPA) receptors, impairing synapse formation and cognitive function [3]. In addition, L-phenylalanine inhibits 3-hydroxy-3-methyglutaryl-CoA reductase, the rate-limiting step of cholesterol biosynthesis and switches forebrain oligodendrocytes to a non-myelinating state, affecting myelination. These mechanisms explain most neuropathological findings of untreated patients, i.e. significantly reduced brain weight, symmetrical reduction of periventricular and cerebellar white matter with vacuolization, and degeneration of the corticospinal tract. Neuronal cell density and even more pronounced axonal sprouting and arborization are strongly reduced and synapse formation rarified.
Newborns with PKU are asymptomatic since fetal L-phenylalanine concentrations are normalized by the mother’s intact metabolism. Untreated individuals, however, almost invariably develop severe cognitive disability with an IQ below 40. The first symptom is delayed development, usually starting around age 3 months, followed by developmental arrest. Epilepsy often presents with infantile spasms. Muscular tone is increased and tendon reflexes are brisk, slowly progressing to spastic para- or tetraparesis. A parkinsonian presentation with tremor is often observed in adults. Affected individuals and their families are confronted with severe behavioral problems and neuropsychiatric comorbidities including autism spectrum disorder, aggressive behaviors, self-mutilation, and anxiety. Constitutional abnormalities such as hypopigmentation of the skin, hair (fair), and iris (blue) develop rapidly due to an impaired metabolism of melanin. Eczematous rash resembling atopic dermatitis is also found and thought to be caused by phenylacetate [10].
The first and still most important therapeutic intervention in PKU is a low L-phenylalanine diet, which should be started immediately after diagnosis, preferably after identification through newborn screening, and continued lifelong [12]. It was estimated that during infancy delayed start of therapy results in irreversible loss of one IQ point per week. There is a worldwide consensus that diet should be started if plasma L-phenylalanine rises above 600 µmol/L; however, there is still controversy about whether untreated individuals whose plasma L-phenylalanine concentrations are constantly between 360–600 µmol/L require treatment or not. Furthermore, there is uncertainty about the target L-phenylalanine levels, in particular during adulthood [7, 13]. With age, tolerance to L-phenylalanine increases, owing to the decreasing vulnerability of the brain. However, adults who do not adhere to diet might be confronted with neurocognitive dysfunction and early-onset dementia. Pregnant women require strict dietary control (L-phenylalanine target range: 120–360 µmol/L) to prevent maternal PKU, an embryofetopathy caused by the teratogenic effects of increased L-phenylalanine concentrations, partially resembling alcohol embryopathy [14]. This includes cognitive disability, microcephaly, brain malformations, congenital heart disease, limb malformation, and/or tracheoesophageal fistula. PKU patients whose PAH activity can be significantly stimulated by its cofactor BH4 should be treated with sapropterin dihydrochloride to increase their L-phenylalanine tolerance [15]. Pegvaliase, a novel enzyme substitution therapy, has recently been approved by the US Food and Drug Administration and opens new therapeutic opportunities for PKU patients.
While individuals who have been treated soon after birth and adhered to recommended therapy have an excellent outcome, late-treated individuals only partially respond to therapy. However, although cognitive disability cannot be reversed, they often show neurological and behavioral improvements, although a strict diet may be difficult to implement in older patients.
Organic Acidurias
Two groups of OADs – “classic” and “cerebral” OAD – have been delineated, based on the clinical presentation. Patients with classic OADs such as methylmalonic aciduria often present in the newborn period or infancy after a short symptom-free interval of days or weeks with life-threatening acute “sepsis-like” metabolic decompensation, including metabolic acidosis, keto- and lactic acidosis, and hyperammonemia. Such metabolic crises can occur at any age and are usually precipitated by catabolism. In contrast to classic OADs, patients with cerebral OADs present with predominant neurological symptoms, which usually develop in the absence of severe metabolic decompensation. Neurological symptoms may manifest acutely such as in glutaric aciduria type 1 or may slowly progress after a variable symptom-free period, typical of L-2-hydroxyglutaric aciduria [1].
Methylmalonic Aciduria Due to Methylmalonyl-CoA Mutase Deficiency
Methylmalonic aciduria (MMA) is the biochemical hallmark of a heterogeneous group of IEMs. This chapter focuses on mutase-deficient MMA, first described in 1967, with an estimated prevalence of 1 in 100,000 newborns. It is caused by bi-allelic mutations in the MUT gene (gene locus: 6p12.3) causing complete (mut0) or partial (mut–) deficiency of the mitochondrial enzyme methylmalonyl-CoA mutase (MUT). MUT requires 5’-deoxyadenosylcobalamin as a cofactor and hence deficient biosynthesis or transport of this cofactor, insufficient dietary intake, or impaired intestinal uptake all result in dysfunctional MUT. MUT converts L-methylmalonyl-CoA to succinyl-CoA in the final step of propionate oxidation, an important anaplerotic mechanism that helps to replenish the Krebs cycle with an important substrate. The amino acids L-isoleucine, L-threonine, L-methionine, L-valine, odd-numbered fatty acids, the side chain of cholesterol, and propionate-producing gut bacteria are major precursors of methylmalonyl-CoA. Biochemically, MUT deficiency results in intramitochondrial accumulation of propionyl-CoA and methylmalonyl-CoA. Since CoA esters cannot cross the inner mitochondrial membrane, there is a systemic accumulation of the name-giving methylmalonic acid as well as 2-methylcitric acid, 3-hydroxypropionic acid, tiglic acid, propionylcarnitine, and propionylglycine [4].
Propionyl-CoA mimics acetyl-CoA and thereby interferes with a variety of metabolic pathways including inhibition of (1) the glycine cleavage system resulting in hyperglycinemia, (2) N-acetylglutamate synthase resulting in hyperammonemia, and (3) pyruvate dehydrogenase complex resulting in lactic acidemia and hyperketosis. In addition, 2-methylcitric acid inhibits the Krebs cycle enzymes, and methylmalonate interferes with succinate transport [16–18]. Furthermore, MUT deficiency causes irreversible dysfunction of an important anaplerotic pathway. The consequence of these converging mechanisms is synergistic impairment of energy metabolism and the mitochondrial part of ureagenesis. Mitochondrial dysfunction as exemplified by the reduced potential of the inner mitochondrial membrane usually activates the PINK1–Parkin system, which then leads to degradation of damaged organelles via autophagy (mitophagy) [19]. Evidence is increasing, however, that mitochondrial quality control through mitophagy is inefficient in individuals with MMA, causing sustained mitochondrial dysfunction. This is demonstrated by a multiple deficiency of mitochondrial enzyme complexes required for oxidative phosphorylation (OXPHOS), particularly cytochrome c oxidase (complex IV), highlighting the fact that acute metabolite-induced mitochondrial dysfunction turns into metabolite-independent organelle dysfunction, with age [20, 21].
Untreated individuals with MMA, mut0 more frequently than mut– patients, present with severe neonatal metabolic decompensation characterized by rapidly developing multi-organ failure, which may be misinterpreted as neonatal sepsis, and biochemically by hyperammonemia, metabolic acidosis, hyperketosis, lactic acidosis, and hyperglycinemia [22]. During infancy and childhood, the brain is at particular risk for basal ganglia damage, mostly affecting the globus pallidus (termed “metabolic stroke”), during severe metabolic decompensations [23]. However, the clinical phenotype is much broader and develops with age. Failure to thrive, developmental delay and intellectual disability, axial hypotonia, extrapyramidal symptoms (mostly dystonia and chorea), seizures, microcephaly, optic atrophy, prolonged QTc interval and cardiomyopathy, metabolic myopathy, pancreatitis, leukopenia, thrombocytopenia, anemia or pancytopenia, and chronic kidney disease all have been described [2, 4]. While hospitalizations due to impending metabolic crises are frequent in infants and children and affect neurodevelopment, these crises become less frequent or even diminish with age and are followed by a chronic progressive disease course with multiple organ dysfunction, resembling OXPHOS disorders [4, 20, 21].
Metabolic maintenance treatment aims to reduce the production of toxic metabolites by sustaining anabolism and dietary restriction of precursor amino acids (L-isoleucine, L-methionine, L-threonine, and L-valine) [4]. As significant propionate production occurs in the gut, intermittent decontamination (10–14 days per month) with oral metronidazole or colistin, as well as measures preventing constipation, are often used. L-carnitine is supplemented with the aim to stimulate physiological detoxification of propionyl-CoA via non-toxic, water-soluble propionylcarnitine and to prevent secondary carnitine depletion. Recurrent hyperammonemia, especially during infancy, may require additional pharmacotherapy with carglumic acid and sodium benzoate [24]. Hydroxy- or cyanocobalamin do not help to stimulate residual enzyme activity in mut0 or mut– patients. Chronic kidney disease often progresses, necessitating hemo- or peritoneal dialysis. To prevent or stop metabolic crises, patients receive emergency treatment including an intensified supply with carbohydrates and carnitine, a transient reduction of dietary protein, and an intensified pharmacological detoxification or extracorporeal removal of toxic metabolites (ammonium, lactate, toxic organic acids) [4]. Overall, the outcome of individuals with MUT-deficient MMA is often disappointing, despite adherence to recommended metabolic therapy. Liver and/or kidney transplantation significantly improves the biochemical phenotype and protein tolerance, but does not reliably protect against neurological complications [8]. Long-term outcome studies are required to understand which patients may benefit from organ transplantation and which transplants – liver, kidney, combined liver–kidney – have favourable outcomes.
Glutaric Aciduria Type 1
Pathological accumulation of glutaric acid, a dicarboxylic acid, is the biochemical hallmark of three etiologically and clinically different IEMs termed glutaric aciduria types 1–3. This paragraph focuses on glutaric aciduria type 1 (GA-1; first described in 1975, which is commonly found with an estimated prevalence of about 1 in 100,000 newborns, but is more frequent (up to 1 in 300 newborns) in some high-risk populations such as the Amish Community in Pennsylvania, USA, and the Oji-Cree first nations in Western Ontario and Manitoba, Canada, due to founder mutations. GA-1 is caused by bi-allelic mutations in the GCDH gene (gene locus: 19p13.13) resulting in deficiency of flavin adenine dinucleotide (FAD)-dependent glutaryl-coenzyme A (CoA) dehydrogenase (GCDH), a mitochondrial key enzyme that catalyzes the oxidative decarboxylation of glutaryl-CoA to crotonyl-CoA in the final catabolic pathway of L-lysine, L-hydroxylysine, and L-tryptophan. As a consequence of GCDH deficiency, glutaric, 3-hydroxyglutaric, and (inconsistently) glutaconic acids as well as of non-toxic glutarylcarnitine accumulate. Due to the limited permeability of the blood–brain barrier to dicarboxylic acids (such as glutaric acid), these strongly accumulate in the brain (so-called trapping hypothesis) [25]. Noteworthy, some of these metabolites are considered neurotoxic. This may explain why individuals with a complete loss of GCDH activity (“high excretors”) have the same high risk of developing neurological disease than those with residual GCDH activity (“low excretors”). Candidate mechanisms are stimulation of excitotoxic pathways via activation of NMDA receptors, inhibition of the 2-oxoglutarate dehydrogenase complex and the dicarboxylate shuttle between astrocytes and neurons, and vascular dysfunction of the brain [16, 26, 27]. In the long run, chronic epigenetic changes caused by enhanced lysine glutarylation of metabolic enzymes and histones may result in alterations of the adaptive response to environmental changes and altered gene expression [28].
Newborns are often asymptomatic but may present with transient neurological symptoms (axial hypotonia, asymmetrical posturing) and macrocephaly, which is found in 75% of patients. Neuroimaging in newborns and infants often reveals hypoplasia of the temporal lobe with subsequently reduced opercularization and widening of the Sylvian fissure. Subependymal pseudocysts and delayed myelination are also commonly seen. All these changes can improve or completely resolve with age in individuals treated early. In addition, subdural fluid collections are occasionally found and are thought to result from increased mechanical vulnerability of bridging veins. These subdural collections can be mistaken as a consequence of non-accidental trauma [29]. The prognostically relevant event of GA-1 is irreversible striatal damage, which may be precipitated acutely by episodes of catabolism or manifest insidiously without apparent trigger. The characteristic time window of striatal injury is usually between 3 months and 36 months of age (peak: 9–10 months). Irreversible striatal injury has rarely been reported after age 6 years. Striatal lesions first manifest in the dorsolateral aspects of the putamen and then spread into a ventromedial direction. The histological hallmark is severe loss of GABAergic medium-spiny neurons, the most abundant neuronal species in the striatum [30]. The caudate and pallidum may also be involved in this process [29]. The consequence of striatal damage is a complex movement disorder with predominant generalized dystonia, superimposed on baseline axial hypotonia. With age, the dystonia tends to evolve from mobile to fixed dystonia. Orofacial dyskinesia is also a consistent finding, resulting in dys- or anarthria, speech apraxia, and impaired swallowing [31]. Less frequently, chorea is the predominant finding. The severity of the movement disorder is best predicted by the extent of striatal lesions. In individuals with severe dystonia, life expectancy is significantly reduced. Fifty percent of severely affected patients die before adulthood due to secondary complications of their movement disorder. In individuals with the high-excretor phenotype, progressive signal changes of periventricular white matter and gray matter (e.g. substantia nigra, nucleus dentatus, and thalamus) as well as subependymal lesions with unclear clinical significance have been reported [29]. This may reflect cumulative neurotoxicity, particularly in untreated patients. Based on its apparently exclusive neurological phenotype, GA-1 had been termed a “cerebral” organic aciduria two decades ago. However, independent of the neurological phenotype, kidney function tends to decline with age and does not appear to be impacted by current therapy, and thus extends the clinical phenotype [5].
Metabolic treatment cannot reverse the neurological phenotype in symptomatic patients once striatal damage has occurred [32]. However, more than 90% of neonatally screened and presymptomatically treated patients who adhered to evidence-based recommendations remain asymptomatic, and symptomatic patients are usually less severely affected compared to the pre-screening era [33]. Recommended therapy consists of a low lysine diet until age 6 years and L-carnitine aiming to stimulate the physiological formation of non-toxic glutarylcarnitine and to prevent secondary carnitine depletion. Since a low lysine diet and carnitine supplementation do not prevent the manifestation of striatal damage during episodes of catabolism, emergency treatment with supplementation of carbohydrates, reduced protein intake, and intensified carnitine supplementation is warranted. After age 6 years, metabolic therapy is liberalized since acute or insidious striatal damage does not develop beyond this age [5]. Animal studies confirmed that these therapeutic measures reduce the accumulation of neurotoxic metabolites [34].
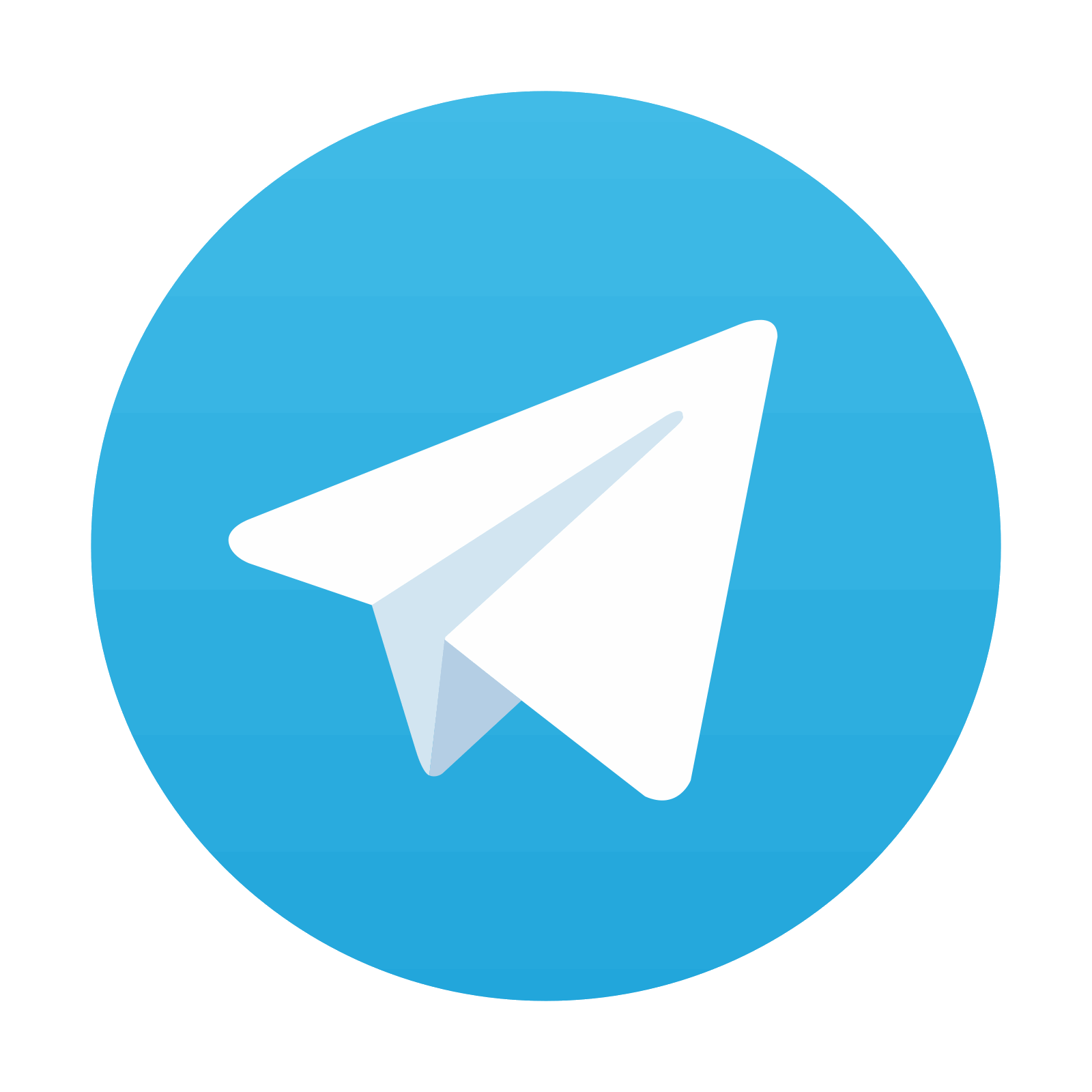
Stay updated, free articles. Join our Telegram channel
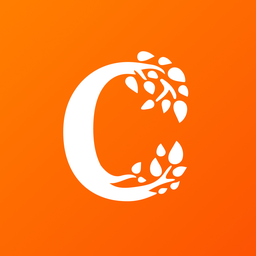
Full access? Get Clinical Tree
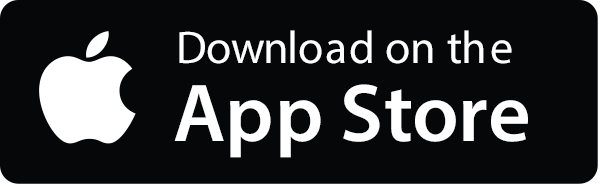
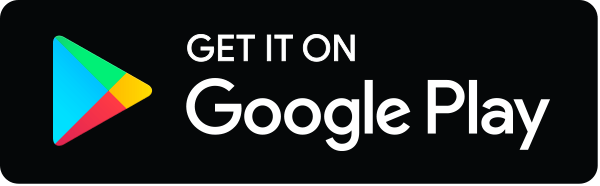
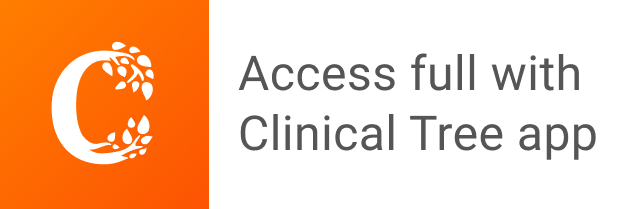