Abstract
Magnetoencephalography (MEG) has emerged as an important tool in the study of mood disorders. Although electroencephalography (EEG) is much more widely utilized, largely due to its low cost and ease of use, MEG has the distinct advantage of enabling accurate localization of brain structures. Although a full discussion of the methodology of MEG is beyond the scope of this brief chapter, we will present a brief overview of the technique and refer the reader to several excellent volumes (1–3) for more information.
15.1 Introduction to Magnetoencephalography
Magnetoencephalography (MEG) has emerged as an important tool in the study of mood disorders. Although electroencephalography (EEG) is much more widely utilized, largely due to its low cost and ease of use, MEG has the distinct advantage of enabling accurate localization of brain structures. Although a full discussion of the methodology of MEG is beyond the scope of this brief chapter, we will present a brief overview of the technique and refer the reader to several excellent volumes (1–3) for more information.
While EEG measures the electric potentials on the surface of the scalp, MEG measures the magnetic fields produced by brain activity. Electric potentials are conducted by the scalp, smearing the signals, while magnetic fields are not attenuated in this way. It is believed that the fields measured by both EEG and MEG reflect the summed local field potentials of large populations of parallel pyramidal cells in the cortex. It is often repeated that MEG is not sensitive to sources oriented radially to the surface of the skull (i.e., on gyri) and can only detect fields from sources parallel to the surface (i.e., sulci). This would only be true were the head a perfect sphere, and for sources that are perfectly radial.
The magnetic fields produced by the human brain are extraordinarily small, on the order of femtotesla. To detect fields of this magnitude, extremely sensitive detectors must be used. Commercial MEG devices make use of superconducting quantum interference devices, or SQUIDs. Briefly, each SQUID is a superconducting loop broken by two insulators, known as Josephson junctions. Small magnetic fields can be detected by SQUIDs because the current flowing in a SQUID will reverse each time a half quantum of magnetic flux passes through the loop. In order to measure the fields, the SQUIDs must be coupled to a flux transformer or a coil sensitive to magnetic fields. There are multiple potential orientations of flux transformers, including magnetometers, and axial and planar gradiometers, each with a different sensitivity pattern. It is a common misconception that MEG systems are insensitive to deep sources. Axial gradiometers have greater sensitivity to deep sources than do planar gradiometers. Numerous studies of axial gradiometer MEG systems have demonstrated the ability to localize activity to subcortical regions, given sufficient signal to noise.
A consequence of the superconducting nature of SQUIDs is that they must be immersed in liquid helium, at 4 kelvin. This makes MEG systems large, cumbersome, and expensive to operate. MEG systems also must be housed in a magnetically shielded room (MSR), made of multiple layers of mu-metal designed to eliminate ambient fields as much as possible. An alternative to SQUIDs are optically pumped magnetometers (OPMs). These devices excite rubidium atoms in a vapor, then measure the opacity of that vapor, which is affected by local magnetic fields. While OPM-based systems have been prominently featured in scientific publications (4), a commercially viable system requires solving issues with sensitivity, the breadth of frequencies that can be measured, and interference of neighboring channels.
Modern MEG platforms utilize hundreds of channels, arrayed more or less evenly around the head. Sophisticated electronics systems are required to translate the reversing currents of the SQUIDs into magnetic field values. Changes in the field are captured by the system at a high frequency in order to obtain measures of brain activity into the gamma range (30 Hz and above). MEG recordings can be captured at rest, or during performance of tasks similar to those administered during functional magnetic resonance imaging (fMRI) exams. Some tasks will evoke responses that are time-locked to the delivery of the stimulus. These evoked response fields (ERFs, in contrast to evoked response potentials, or ERPs, measured by EEG) can be compared between diagnostic groups or task condition in terms of their amplitude or latency to peak. Other tasks may induce changes in oscillatory power, which can be compared between groups or task conditions. These induced power changes may occur in the canonical frequency bands, including delta, theta, alpha, beta, and gamma. Different frequencies of oscillation have different mechanisms and functional significance, and some are better understood than others; an in-depth treatment on this subject can be found in Buzsaki’s Rhythms of the Brain (5).
While MEG signals can be analyzed without source localization (referred to as “sensor space”), the power of MEG comes from transforming the signals to the space of the subject’s brain, usually with the addition of a high-resolution MRI. There are multiple ways to project MEG data into source space, and all are approximations. Some methods localize a small number of sources with high accuracy, which may work well when dealing with simple sensory inputs (i.e. localizing the response to an auditory stimulus to auditory cortex). Other methods will produce maps of the fields measured at all points on the surface of the cortex or within the full brain volume. Broadly speaking, these algorithms generally require minimization of a measure of overall power. An excellent discussion of methods for projection into source space can be found in MEG: An Introduction to the Methods (1).
15.2 MEG in Mood Disorders
Although the application of MEG to the study of mood disorders has been steadily increasing, there are still less than 100 peer-reviewed studies. Unfortunately, most of these studies have small sample sizes, and the majority utilizes medicated patients, complicating generalization and interpretation. We will review most published works here, in order to inform the reader of the full extent of the literature. Most studies involve patients with major depressive disorder (MDD), although there is a growing literature on bipolar disorder (BD). We will cover the studies by topic, beginning with studies involving sensory evoked fields. We’ll move on to more complex task-based studies, including those investigating emotional processing, as well as resting-state studies. Finally, we will cover the field of neuromodulation treatments and MEG.
15.3 Sensory Evoked Fields
Synchronized, time-locked responses are evoked in primary sensory cortices in response to a multitude of stimuli – robust visual, auditory, motor, and somatosensory fields can be measured and localized with MEG. These fields are of particular interest in mood disorders because they are measures of neuronal excitability, and thus may reflect synaptic plasticity. As mood disorders are increasingly characterized as disorders of homeostatic plasticity, interest is growing in interventions which result in synaptic potentiation, which can be measured by pre- versus posttreatment measures of sensory evoked responses. Furthermore, underlying abnormalities in basic cortical sensory responses may reflect global alterations in brain function, rather than system/circuit level dysfunction.
15.3.1 Motor and Somatosensory Evoked Fields
Somatosensory evoked fields (SEFs) are generated in somatosensory cortex in response to median nerve stimulation at 20 and 30 ms poststimulation. Although one study found reduced M20 amplitudes in MDD compared to healthy controls (6), another small study in a partially euthymic group reported no differences (7). The idea that neuronal excitability may be attenuated in MDD is consistent with a series of studies examining the SEFs to tactile stimulation and treatment with ketamine. First, Cornwell et al. (8) found that patients with MDD who responded to ketamine showed an increase in the evoked gamma band response to a somatosensory stimulus; nonresponders to ketamine showed no increases. A follow-up study in both MDD and healthy subjects using a placebo control found no differences in groups at baseline, but an increase in the evoked gamma response in responders, and a linear relationship between response and the difference in evoked gamma power between ketamine and placebo conditions (Figure 15.1a and b)(9). Basic sensory tasks are also ideal for analysis using dynamic causal modeling (DCM). DCM uses Bayesian priors to estimate structural models given endogenous interregional connections, modulatory connections influenced by task state, and a driving stimulus. Indeed, a DCM analysis of these data revealed that post-ketamine, NMDA-mediated backward connections were elevated in MDD compared to healthy subjects (Figure 15.1 c–e)(10). The enhancement of backward connections post-ketamine in MDD is consistent with the idea that successful antidepressant treatment enhances prefrontal modulation of basic sensory and limbic processes. In total, these studies not only provide more evidence for reduced neuronal excitability in MDD, but also demonstrate that synaptic plasticity may be a crucial mechanism of action of ketamine, and possibly other antidepressants.
Figure 15.1 Panel (a) shows averaged evoked response fields from contralateral somatosensory cortex for left- and right-handed stimulation. The increase-evoked response post-ketamine is evident in patients who respond to ketamine (MDD-R) but not nonresponders (MDD-NR) or healthy subjects (HC). Panel (b) illustrates that a large increase in peak gamma power between ketamine and placebo sessions is associated with a favorable antidepressant response to ketamine. Panel (c) illustrates the DCM model including multiple cell layers, and (d) illustrates the specific model used incorporating bilateral prefrontal cortex (Fr) and primary somatosensory cortex (S1), along with a driving input. Panel (e) shows the between-subjects difference in NMDA-mediated connectivity post-ketamine infusion in the backward connection from right Fr to S1 (dashed line in panel d). Figure modified from previously published work (10)
15.3.2 Auditory Evoked Fields
Auditory stimuli evoke a field at approximately 100 ms poststimulus (M100), generated in Heschl’s gyrus. Smaller auditory evoked fields (AEFs) are also generated at approximately 50 ms (M50) and 200 ms (M200) poststimulus (11); other fields may be generated by more complex task designs. While many studies have found alterations in early auditory processing in mood disorders, the available results do not converge on a single, reliable marker for MDD or BD. In MDD, decreased latency (12) or no differences (13) in M100 have been reported; in BD, one study found reduced M100 and M200 fields bilaterally compared to healthy subjects (11), although another study found no differences in the M100(14, 15). Notably, it has been hypothesized that the sensitivity of the M100 and M200 to stimulus intensity may be related to serotonergic function (16), making studies in medicated patients particularly problematic.
One widely used class of auditory paradigms are “oddball” tasks. Classically, these tasks present a series of tones, most of a single frequency, with intermittent tones presented at a different frequency. The mismatch negativity (MMN) is derived by subtracting the evoked field of the frequent event from that of the rare event, typically producing a negative deflection. MMN is thought to represent processing that occurs before conscious attention to the stimulus (14). Lower MMN amplitudes have been reported in MDD (13, 17) and BD, with amplitudes of MMN in BD correlated with a measure of mania (18). Shorter latencies to MMN have also been reported in MDD (17), with longer latencies observed in BD (14), potentially indicating a possible diagnostic marker. At least one study found no changes in MMN in MDD (12).
Periodic stimuli, either oscillating tones or click-trains, elicit an auditory steady-state response (ASSR) synchronized to the stimulus in phase and frequency. Patients with BD have demonstrated significantly reduced ASSR amplitudes and phase locking values to gamma frequency stimuli (19, 20). Interestingly, MDD patients demonstrated significantly greater ASSR amplitudes than BD patients, with controls falling between MDD and BD patients (although MDD patients did not significantly differ from healthy volunteers). It is thought that ASSR reflects the efficiency of GABAergic interneuron activity in response to rhythmic stimuli (20). Previous reports have related decreased ASSR to increased spontaneous gamma power (21), although none of the studies reviewed here examined spontaneous gamma power in auditory cortex. Nevertheless, if the greater ASSR amplitudes in MDD are reflective of lower basal gamma power, this would be consistent with findings from MEG and EEG that increasing gamma power in patients with MDD using either ketamine (22) or TMS (23) will relieve symptoms of depression. Likewise, there is some evidence from EEG that gamma power is elevated in BD (24).
Although other auditory paradigms have been employed, the evidence is insufficient to support firm conclusions. Studies investigating the typical left-right asymmetry in the location of either M100 evoked responses (15) or ASSR (25) find evidence for reduced asymmetry in BD subjects, potentially indicating alterations in brain development. Paired stimulus paradigms where two stimuli (S1 and S2) are presented can be used to study inhibitory gating mechanisms, whereby an initial stimulus invokes inhibitory mechanisms, attenuating the response evoked by the second stimulus. Increased M50 responses to S2 in BD compared to controls been found, along with greater S2/S1 ratios for both the M50 and M100(26). This may indicate a failure in sensory gating by inhibitory neuronal pathways, potentially resulting from neuronal hyperexcitability (26). Auditory stimuli can also induce coherent oscillations, and there is evidence that patients with BD demonstrate increased evoked power in the beta and gamma ranges, at least in response to speech sounds (27).
Taken together, the literature examining basic sensory processing in mood disorders points toward systemic dysfunction. There is evidence from auditory, motor, and somatosensory studies supporting the notion that neuronal excitability is attenuated in MDD as compared to healthy subjects. In contrast, multiple studies in BD suggest an increase in neuronal excitability, although results are inconsistent, potentially due to medication effects or effects of current mood state (i.e., manic vs. depressed). These abnormalities in basic sensory processing are significant, because they implicate fundamental neurophysiological processes, rather than deficits in specific monoaminergic neurotransmitter systems or isolated functional networks and/or brain regions.
15.4 Emotional Paradigms
Given that mood disorders are clinically disorders of emotion regulation, numerous studies have compared patient groups in both behavioral and neurophysiological responses to emotional tasks. As in fMRI, many paradigms have been used, and responses to similar paradigms may be quite different. Evoked response paradigms typically measure modulation of either visual evoked fields (VEFs) or evoked response fields to faces (M170) by the affective valance of the stimulus. Induced oscillations in response to more complex emotional tasks can also be examined. The primary limitations of these studies are the lack of replication, especially given that studies with slightly different paradigms may produce discrepant results.
15.4.1 Evoked Responses to Affective Stimuli
Several studies have utilized the VEF to emotionally arousing visual stimuli to examine a potential bias toward negative stimuli and modulation of affective arousal systems. In a study of twenty-five healthy volunteers and twenty-five unmedicated patients with MDD, subjects showed significantly attenuated VEFs in response to emotional stimuli across the parietal cortex, and stronger VEFs to negative rather than positively valanced images (healthy subjects showed a trend toward greater response to positive stimuli). Both the VEF amplitude in the right temporoparietal junction and the difference in VEF amplitude to negative vs. positive stimuli in the dorsolateral prefrontal cortex (DLPFC) were correlated with depression severity. A subset of fifteen patients underwent repeat scanning after treatment with mirtazapine and showed partial normalization of the parietal VEF, although the neural bias toward aversive stimuli was unchanged (28). A similar negative bias was observed in the occipital M300 response to faces in an affective oddball-type paradigm in dysphoric patients, some with a diagnosis of MDD (29). Affective stimuli can also be subjected to intensity modulation to evoke rhythmic steady-state visual evoked fields (ssVEFs). Similar to prior findings (28), MDD patients exhibited attenuated ssVEF amplitudes compared to healthy subjects in right temporoparietal cortex, although with reduced modulation by valance rather than a negative bias (30). A replication study demonstrated that this effect may have been driven by patients who had a family history of mood disorders (31). These results are consistent with the findings of reductions in evoked fields in other sensory modalities, as well as substantial behavioral and fMRI evidence supporting a bias toward negative stimuli in MDD (32).
Another type of task utilized in fMRI involves repeated presentation of emotional stimuli to examine habituation, the normal decrease of neuronal activity in response to a repeated stimulus. Unmedicated MDD patients demonstrated increasing amplitudes of the evoked responses to repeated negative faces in the pregenual area of ACC (pgACC), while healthy subjects showed decreasing amplitudes; this was true only for the first of two face sets presented. The change in both pgACC and amygdala activity correlated with subsequent antidepressant response to ketamine (33).
Several studies carried out by the same research group examined a task utilizing short videos of emotional facial expressions, where subjects were asked to respond if the expression shown was sad. Data were analyzed using several different methods for connectivity, including DCM (discussed earlier), Granger causality, and coherence. Granger causality calculates directed connectivity by modeling the signal in a given region as predicted by the signal in other regions at earlier time points. Coherence can intuitively be interpreted as a version of correlation including the dimension of frequency. Overall, these studies pointed to the importance of the amygdala in network models, and generally found enhanced connectivity from amygdala to prefrontal cortical areas, and decreased connectivity from prefrontal cortex to amygdala in MDD patients compared to controls (34–36). One additional study using the same paradigm and both MEG and DTI found that coupling between the salience network and ventral attention network was elevated in depressed patients compared to controls (37). In total, these results echo the simpler affective VEF results, suggesting a bias toward negative stimuli, as well as the importance of the ACC and amygdala in emotional face processing. These results also support the notion that depression involves impaired top-down control of the limbic system by prefrontal areas.
15.4.2 Induced Oscillatory Responses to Affective Stimuli
Few studies have been performed examining induced changes in power in response to emotional paradigms in MEG, despite the obvious parallels to fMRI paradigms. One study used the above-described emotional identification task (34–36), and applied a multichannel matching pursuit (MMP) algorithm to extract the principle signal elements, from which oscillatory power amplitudes were calculated. These values were used to determine if a support vector machine could discriminate MDD patients from healthy subjects. Elevated alpha and beta power, primarily in frontal and central sensors, was found to provide the greatest discriminatory power, though reduced theta power was also noted (38). An implicit version of this task has also been employed, where subjects respond with the gender of the face rather than the valence. While both MDD and BD patients exhibited focal areas of increased alpha power in response to angry faces, with some regions discriminating between groups, these regions did not map onto specific known functional networks (39). A study using the same paradigm, but collapsing over all stimulus emotions, found reduced gamma power in both MDD and BD compared to controls in widespread brain areas, with activity in parieto-occipital sensors differentiating MDD from BD subjects (40). Unfortunately, the lack of convergent evidence from these studies limits their broader interpretation.
15.4.3 Other Cognitive Tasks
Numerous cognitive domains are impaired in mood disorders, and several studies have used cognitive tasks and MEG to elucidate the neural mechanisms behind these deficits.
Reduced hippocampal volume is one of the most robust structural findings in MDD (41), motivating studies of spatial navigation, a domain in which the hippocampus plays a vital role. A virtual Morris water maze navigation task was administered to nineteen unmedicated patients with MDD. Behaviorally, the MDD patients showed longer latencies to find a hidden platform than healthy subjects. Hippocampal theta activity during hidden platform searching was significantly lower in the depressed subjects, and parahippocampal cortex theta power was correlated with longer latency to navigate to the platform (42).
Working memory processes are also disrupted in mood disorders, and a study of patients with MDD found that beta desynchronization in response to increasing task load in the sgACC and pgACC was associated with subsequent antidepressant response to ketamine. Patients who showed the greatest desynchronization, which may be associated with greater neuronal engagement, were most likely to respond to ketamine (43). Notably, the region identified overlapped with previous findings using an emotional processing task (33, 43). Furthermore, connectivity between the pgACC and the amygdala was negatively correlated with antidepressant response (43). A follow-up study showed that differences in beta power between healthy and MDDs were driven by the subset of patients who had significant anxiety. These anxious depressed patients showed greater beta power in the cuneus/precuneus, insula, and inferior and middle frontal cortex during the highest cognitive load condition (2-back) compared to the easier condition (1-back)(44).
As with the emotional processing results, MEG results from cognitive processing studies are difficult to interpret due to the relative lack of replication data. However, the MEG findings are consistent with the well documented behavioral effects, and these preliminary data suggest that MEG may provide information beyond that offered by fMRI, in that the added dimension of frequency may better pinpoint what neuronal processes are responsible for behavioral dysfunction.
15.5 Resting-State MEG
Due to the inherent richness in electrophysiological signals, a broad array of metrics can be used to describe the resting state in MEG. In addition to measures of spectral power, there are a variety of linear and nonlinear metrics, as well as connectivity metrics. While some measures have been used to derive resting-state networks similar to those seen in fMRI, other measures derive features unique to electrophysiological networks. While the demonstration that the canonical fMRI resting-state networks can be observed in MEG data has elevated the profile of MEG in the greater neuroimaging community, it is our contention that MEG data provide crucial validation for the fMRI findings, rather than the other way around. While the fMRI signal represents a neuronal signal convolved with a hemodynamic response function, MEG data are a direct measure of neuronal function. Thus, MEG may be interpreted as closer to the ground truth than other methods, despite the relatively lower spatial resolution.
15.5.1 Spectral Power
Although few studies exist that examine only spectral density, many resting-state studies include an examination of power in the canonical bands, most frequently in sensor space. The reader may know that alpha asymmetry is one of the most widely reported EEG findings in MDD; this value is unreliable in MEG, since apparent asymmetry may result if the cortex is not perfectly centered in the imaging device. We will discuss each canonical frequency band later, though the frequency ranges for each band are not standardized and differ slightly across studies.
Although studies of infra-slow wavelengths exist, most studies consider delta (2–4 Hz) to be the lower end of physiological oscillatory processes. Delta waves dominate during deep sleep; prominent delta waves during waking generally indicate serious neurological dysfunction. Frontal delta may be corrupted by artifacts from eye blinks or movements, which is particularly problematic when studying connectivity. Although source reconstruction methods should localize the artifact to the eyes, there is inevitably some signal leakage and results in orbital cortex should be interpreted with caution. Elevated delta activity, correlated with severity, has been reported in MDD as compared to healthy subjects over the right occipital cortex (45), while another study showed elevated 2–6 Hz activity over the left hemisphere (46). Elevated delta power has also been observed in BD posteriorly (47). Theta oscillations (4–8 Hz) are also considered slow waves and their measurement can also be corrupted by eye blinks or movements. Reductions in theta power in MDD compared to controls has been noted in frontal areas (48) as well as in occipital parietal and right temporo-central regions (49); elevated theta has been noted in posterior sensors in BD compared to healthy subjects (47).
Alpha oscillations (8–12 Hz) are the most prominent in the human brain, particularly when the eyes are closed, and are hypothesized to be related to the default mode network. Reduced parietal alpha has been noted in MDD as compared to healthy subjects, with decreased alpha associated with greater depressive symptoms (48). Desynchronization of beta frequency oscillations (12–30 Hz), resulting in reduced beta power, has been associated with attention and cognitive demands. At rest, stronger frontal and parietal beta power has been observed in MDD compared to healthy subjects (48).
Gamma oscillations, at frequencies above 30 Hz, are generated through recurrent inhibition in networks of GABAergic interneurons, or through feedback networks of GABAergic interneurons and parvalbumin-expressing glutamatergic pyramidal cells (50). Gamma oscillations are reflective of inhibition/excitation balance and may be a proxy measure for synaptic homeostasis (51). Despite the importance of these oscillations, few studies have directly measured basal gamma power in patients with mood disorders, surprising given the mounting evidence that alterations in homeostasis underlie depression (52). Several EEG studies have reported increased gamma power in MDD (53, 54) and BD (24), although MEG studies in both BD (47) and MDD patients (22, 48) have failed to find significant differences relative to controls. Gamma power oscillations are readily contaminated by muscular artifacts, which may be particularly problematic in EEG recordings where spatial localization is usually not performed. Once MEG data have been projected into source space, artifactual increases in gamma power due to muscular activity will be readily apparent along the edges of temporal cortex (due to muscular activity in the jaw) and cerebellum (due to muscular activity in the neck).
The rapid acting antidepressant ketamine has been shown to produce robust increases in gamma power in both animal models and in MEG studies in healthy subjects (55, 56). A study in both patients with MDD and healthy controls showed that gamma power is increased in widespread areas across the cortex up to 6–9 hours post ketamine infusion (Figure 15.2a–c)(22). Elevated gamma power hours after the acute infusion may reflect glutamatergic modulatory activity of an active metabolite of ketamine (2 R,6 R; 2S,6S Hydroxynorketamine, or HNK). The relationship of the increase in gamma power to the antidepressant response is not linear, however, and there is evidence that basal inhibition/excitation balance is a key factor. Indeed, post-ketamine infusion gamma power demonstrated a significant interaction of baseline gamma and the antidepressant response to ketamine. Patients with low baseline gamma power demonstrating large increases in gamma power post-ketamine tended to show favorable antidepressant responses; patients with high baseline gamma power demonstrating large increases in gamma power post-ketamine tended to show poor responses (Figure 15.2e)(22). These results provide further support for alterations in homeostatic regulation in MDD, and potentially point to gamma oscillations as a fundamental metric of homeostasis.
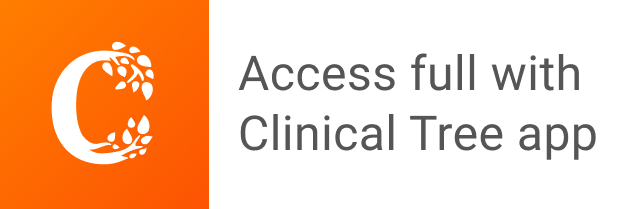