Chapter 19 Histopathology findings in MRI-negative focal epilepsy
MRI-Negative Epilepsy, ed. Elson L. So and Philippe Ryvlin. Published by Cambridge University Press. © Cambridge University Press 2015.
Most surgically resected epileptogenic brain lesions are detectable by high-resolution MRI at 1.5 or 3 T, such as long-term epilepsy-associated tumors and hippocampal sclerosis of malformations of cortical development (MCD), and MRI visibility is a favorable predictor of postsurgical seizure control. However, recent studies suggest that comparable seizure control can be achieved after epilepsy surgery in patients with MRI-negative TLE, with 55% of patients remaining free of disabling seizures after a mean follow-up of 2 years [1] to 5.8 years [2]. In this chapter, we will discuss the spectrum of histopathological changes that can be observed in tissue specimens obtained from patients with MRI-negative focal epilepsy, with particular emphasis on the cellular architecture that hides such lesions from detection with currently available MRI protocols. However, neither the European Epilepsy Brain Bank nor the German Neuropathology Reference Center for Epilepsy Surgery allow us yet to reliably retrieve clinical information of how many operated patients were reported MRI negative. The increasing interest in this matter will help us to close this knowledge gap in the near future.
MRI-negative FCDs
The most prominent example for a structural brain abnormality identifiable in MRI-negative focal epilepsies may be focal cortical dysplasia (FCD) type IIa (Figure 19.1); FCDs represent a composite group of cortical malformations [3], which are increasingly recognized as a frequent morphological substrate for severe therapy-refractory epilepsy in children and young adults. However, not all FCD variants can be reliably detected by high-resolution MRI [4; 5]. The term FCD was originally coined by Taylor and colleagues in 1971 describing ten patients with microscopic evidence for dysmorphic neurons [6]. In half of these patients, the authors also described balloon cells. The current ILAE classification separate both variants into FCD type IIa (dysmorphic neurons, no balloon cells) and IIb (dysmorphic neurons and balloon cells) [3]. Yet, there is no distinguishing evidence for the clinical course, etiology and molecular-genetic or biological pathomechanisms of type IIa and IIb FCDs [5]. Furthermore, dysmorphic neurons are very similar between both variants and cannot be distinguished by morphometric analysis [7]. It is solely the presence of balloon cells, accompanied by lack of myelin and oligodendrocytes that makes the difference between the two subtypes of type II FCDs.
Figure 19.1 MRI findings in histopathologically verified FCD type IIa. Presurgical MRI findings (T2) at 1.5 T (A) and 3 T (B) and postsurgical 3 T (C) in a patient with histopathologically classified FCD type IIa. D: NeuN staining revealed an abnormal layering with intermingled dysmorphic neurons. E: Dysmorphic neurons always present with an abnormal accumulation of neurofilament proteins (SMI32 staining). Red dotted line in C indicates the extent of resection in the left temporal lobe. Roman numbers in D: cortical layers. Insets in D and E: higher magnification showing a typical dysmorphic neuron. Scale bar in E = 500 µm; applies also to D. Scale bars in insets in D and E = 50 µm.
We recently studied a cohort of 52 FCD patients using the 2011 classification of the International League Against Epilepsy (ILAE) and systematically analyzed those histopathological characteristics which could also be assessed on MRI, i.e., cortical thickness, gray–white matter boundary, myelination and cellular composition of FCD subtypes [7]. In contrast to existing data, cortical thickness was significantly increased in both FCD type II variants, with a distinct loss of myelin content specifying the “transmantle sign” of FCD type IIb [7]. Presence of the transmantle sign has been suggested to allow complete resection of FCD type IIb and achieve seizure control in up to 80% of patients [5]. In contrast, FCD type IIa is less frequently encountered and more likely to escape visually guided MRI reading, even in highly experienced centres [5]. Abnormally increased cortical thickness in FCD type IIa lesions, as well as abnormal gray–white matter boundary due to increased neuronal heterotopia, could be detectable by systematic use of modern morphometric post-processing MRI protocols [8]. However, cortical thickness is always difficult to assess on MRI unless very thin sections are acquired as a volumetric data set. Prospective imaging–histopathology correlations will be, therefore, essential to further improve our knowledge of disease-specific cellular components and their corresponding MRI signaling abnormality.
Another challenge in presurgical MRI diagnosis concerns FCD ILAE type I. Systematic histopathological analysis identified a smaller cortical ribbon with higher neuron densities and persisting neuronal microcolumns in FCD type Ia [7, 9, 10]. The 2011 ILAE classification introduced this new FCD type Ia because it likely represents a specific clinicopathologic entity [3]. Patients with such FCD are characterized by early seizure onset, severe psychomotor retardation and mild hemispheric hypoplasia without MR visibility of any other lesion. Drug resistance is frequent and surgical resection-achieved seizure control in only 21% of children in an initial series addressing this isolated FCD subtype [11]. With increasing diagnostic and neurosurgical experience, however, the prospect for this peculiar group of young patients to become seizure-free has improved to almost 50% of cases [12]. Indeed, the vast majority of FCD type I patients from our European Epilepsy Brain Bank (EEBB) series belong to this clinicopathologic entity. Neuropathological hallmarks include abnormal cortical layering affecting both radial migration and maturation of neurons. This aberrant pattern resembles those neurodevelopmental minicolumns described by the “radial unit lineage model” [13]. A minicolumnar organization of the neocortex, especially in somatosensory regions representing visual and auditory fields and the magnopyramidal region of the temporal lobe, has been described in normal and diseased brain [14; 15; 16; 17]. However, to date, there are no molecular–biological nor genetic data available to clarify or even suggest the underlying pathomechanism in this difficult-to-treat-epileptic disorder. In contrast to FCD type Ia, FCD ILAE type Ib presents with abnormal cortical architecture compromising its horizontal layering. Neither specific imaging findings, clinical courses nor histopathology patterns have been systematically described for this rare disorder [7], and it is most likely that current MRI protocols will not reliably detect this FCD.
MRI-negative hippocampal sclerosis
Five to 10% of histopathologically confirmed TLE patients with hippocampal sclerosis (HS) do not present with classical MRI abnormalities, which include hippocampal atrophy and increased intensity on T2-weighted or FLAIR images (Figure 19.2). This results from the heterogeneous presentation of clinicopathological HS subtypes, i.e., including a rare and atypical variant with predominant neuronal cell loss in CA4 only, also known as HS ILAE type 3 [18, 19].
Figure 19.2 MRI findings in histopathologically verified type 3 of TLE-HS. A: Presurgical T2 MRI finding (3 T) in a TLE patient with histopathologically classified hippocampal sclerosis on the right side (white arrow). Here, volumetric loss was virtually not detectable. B: NeuN staining confirmed the diagnosis of HS ILAE type 3 showing a neuronal cell loss predominantly affecting sector CA4 [19]. CA1–CA4: Sectors on the cornu ammonis. DGe: External limb of the dentate gyrus. DGi: Internal limb of the dentate gyrus. Scale bar in B = 1000 µm.
Hippocampal sclerosis is the most frequent structural brain lesion encountered in adult patients with drug-resistant epilepsy, and surgical resection is an established treatment modality [20] offering postoperative seizure freedom within the first 2 years in approximately 60–80% [21; 22; 23; 24; 25; 26]. Clinical studies define mesial temporal lobe epilepsy, however, as a heterogenous entity with different etiologies and clinical histories [27; 28; 29; 30]. Neuropathological investigations have also described different patterns of neuronal cell loss within hippocampal subfields [31; 32; 33; 34; 35; 36; 37; 38; 39; 40]. The earliest neuropathology study in epilepsy patients dates back to 1825, in which Bouchet and Cazauvielh described a hardened and shrunken hippocampus in autopsy brains from patients with clinical history of epilepsy [41]. In 1899, Bratz made available a detailed description of unilaterally atrophic hippocampus, illustrating severe loss of pyramidal neurons and gliosis in CA1, less severe neuronal loss in the hilus of the dentate gyrus and adjacent sector CA3, and preservation of neurons in the CA2, subiculum and the granule cell layer of the dentate gyrus [41]. In 1966, Margerison and Corsellis defined two types of hippocampal damage [18]. One was similar to that from Bratz [42] with severe neuronal loss in CA1 and CA4, and sparing of CA2. The other pattern was characterized by neuronal loss confined to the CA4 region or “end-folium,” termed “end-folium sclerosis.” Since then, atypical HS variants have been histopathologically confirmed and can be detected in up to 16% of all surgical TLE cases [33, 39, 39, 40]. With the continuous progress of imaging techniques in terms of field strength and post-processing, it can be expected that histological knowledge of HS subtypes will translate into clinical protocols helping to recognize such atypical HS variants already during the presurgical clinical monitoring. Indeed, the degree of atrophy has been shown to correlate with the severity of neuronal loss within hippocampal subfields as determined by various pathological gradings, including Wyler’s classification [43, 84]. However, the limited resolution of current clinical protocols still precludes a direct visualization of hippocampal subfields on MRI.
Another intriguing issue remains the association between HS and FCD, classified as FCD ILAE type IIIa [3]. Despite the many published results, neither a distinct etiology nor a clinicopathological phenotype for the association between HS and FCD IIIa has been identified, which elicits continuous debates about the issue [44]. Notwithstanding, HS is frequently associated with other pathologies [45], and electroclinical as well as imaging abnormalities in TLE–HS patients often extend beyond the hippocampus, suggesting a more widespread substrate for the generation or persistence of seizures [46; 47; 48; 49; 50]. Ipsilateral temporal atrophy with temporo-polar gray/white matter blurring can be visible on MRI in up to 70% of TLE–HS patients [51; 52; 53]. It was often regarded as a sensitive radiological FCD marker, but a recent study directly correlating 7T MRI with histopathology including electron microscopy revealed severe and patchy myelin loss in temporal white matter as underlying substrate of the abnormal MRI signal, rather than any kind of cortical/subcortical dysplasia [54]. In fact, histopathologically proven cortical abnormalities in TLE-HS patients are rare and cannot be reliably detected by current MRI protocols. In only 10% of surgical specimens from temporal lobe of HS patients, an abnormal band of small neurons can be observed in the outer part of neocortical layer II [55]. This pattern presents with severe neuronal cell loss in layers II and III and laminar astrogliosis. There is no correlation between this FCD IIIa variant and MRI findings [56, 57]. Small “lentiform” nodular heterotopia can be identified as another structural abnormality in the temporal lobe of patients with TLE–HS, which also remain undetected by MRI [58]. However, we do not have evidence for the dysplastic nature of these MRI invisible HS-associated lesions nor for their pathophysiologic impact to trigger seizures. Several aspects argue rather for a common etiology between HS and FCD type IIIa. Patients from both groups have a similar age at onset and a similar history of febrile seizures as an initial precipitating injury [59]. No other clinical differences have yet been identified between HS and HS/FCD type IIIa cases [54]. Accordingly, postsurgical outcome is similar in patients with HS only compared to HS with FCD type IIIa [60].
MRI-negative abnormalities in white matter
Increased numbers of heterotopic white matter neurons can be frequently encountered in epileptic brain tissue (Figure 19.3), and was described in children and adults with hippocampal sclerosis, FCDs as well as in patients with primary generalized epilepsy [61, 62, 63, 64, 7]. However, this cellular abnormality is usually not visible at the MRI level (Figure 19.3A) and does not account for a blurred white-gray matter boundary visible in almost 70% of patients with TLE–HS [54]. Heterotopic neurons in the white matter even represent a physiological feature of the temporal lobe [65, 66, 67]. Their significant increase and abundance in epileptic tissue suggest, however, an association with chronic epileptic activity, i.e., seizure-induced neurogenesis [68] or may point to early disturbances in the architectural development of the cortex and white matter [65]. According to the current ILAE classification of FCDs, increased numbers of heterotopic neurons in white matter location should be neuropathologically diagnosed as a mild form of cortical malformation (mMCD type II) using Palmini’s classification system [68], if occurring as isolated finding without HS, tumors or other principal lesions [3].
Figure 19.3 MRI findings in histopathologically verified mild malformation of cortical development (mMCD type II). Presurgical (A) and postsurgical (B) MRI findings (T2) at 3 T in a patient with histopathologically classified mMCD type II. NeuN (C) and Map2 (D) stainings reveal a normal thickness of the cortical ribbon without gross architectural disturbances. Note the blurred gray-white matter boundaries (white arrows in C and D) and the increased numbers of heterotopic neurons. within the subcortical white matter (WM). Red dottet line in B indicates the extent of resection in the right temporal lobe. Roman numbers in C: cortical layers. Scale bar in D = 500 µm applies also to C.
On the other hand, MRI abnormalities are frequently encountered in white matter of epilepsy patients, but usually lack any histopathological counterpart. It rather suggests a relationship between seizures and transient functional white matter changes. MRI scans performed early after status epilepticus identify temporary occurrence of brain edema [70, 71], which could also be experimentally induced following kainate injection [72]. In agreement with these findings, seizure activity induces local vasodilatation and enhanced cerebral blood flow. Other pathophysiological consequences of seizure activity include enhanced metabolic activity followed by consumptive hypoxia [73] as well as a transient rise of intracerebral pressure, which is rapidly normalized via blood autoregulation mechanisms. The latter can be impaired by hypercapnia which also results in focal breakdown of the blood–brain barrier (BBB) and extravasation of protein and liquid into the brain [74]. Thus, abundance of substances from blood plasma within perivascular spaces supports severe white matter BBB dysfunction and may directly induce an epileptogenic focus [75]. Indeed, angiopathic white matter changes with a significantly enlarged Virchow–Robin space have been histopathologically described in human epilepsy brain tissue [76].
MRI-negative and microscopically nonlesional
In our large European Epilepsy Brain Bank series, about 8% of all specimens were microscopically reported negative or nonlesional. Microscopy negative cases may result from off-target neurosurgery or insufficient tissue sampling. However, we will have to also consider subtle microscopic lesions beyond currently available detection levels. Another issue of debate remains the presence of reactive astrogliosis, which is a consistent finding in any epileptogenic brain tissue. To date, astrogliosis is not classified as specific neuropathologic disease entity. Astrogliosis can be microscopically detected by immunostaining for glial fibrillary acidic protein (GFAP), and presents with a broad spectrum of changes ranging from reversible cell hypertrophy with preservation of cellular domains to long-lasting scar formation with rearrangement of tissue structure [77]. Since astrogliosis is also common in any other neurological disorders without seizure, we assume astrogliosis is a consequence of chronic epileptic activity rather than a specific epileptogenic trigger. This view has been challenged by the many discoveries on pathophysiological mechanisms elicited by astroglia [78]. As a prominent example, the molecular phenotype of astrocytes significantly influences neuronal microenvironment and contributes to increased neuronal excitability [79]. The contribution of astrocytes to proepileptogenic inflammatory signaling cascades is also well recognized [80]. In addition, astrocytes are the brain’s resource for adenosine-kinase, a degrading enzyme for adenosine [81]. Experimental data showed evidence that any focus of astrogliosis will create adenosine deficiency, and, thereby, promote a proictogenic microenvironment [82, 83]. Describing and clarifying these diverse cellular reactivity patterns in epileptic tissue at a clinical diagnostic level, as well as deciphering their epileptogenic cellular and network properties, represent an intriguing field of translational research in the future.
References



















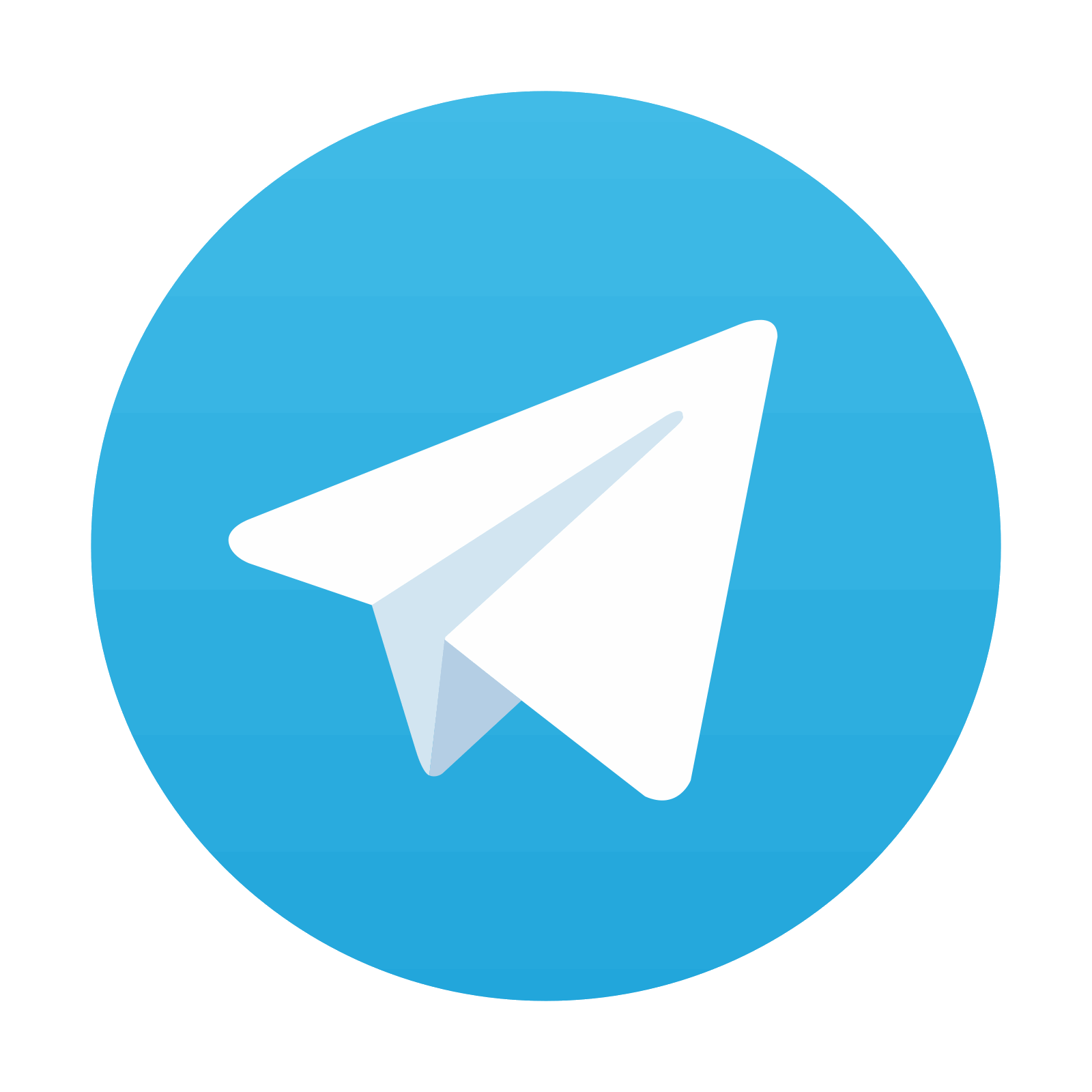
Stay updated, free articles. Join our Telegram channel
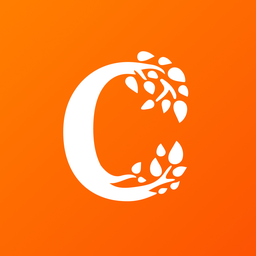
Full access? Get Clinical Tree
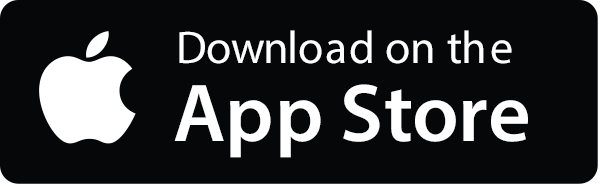
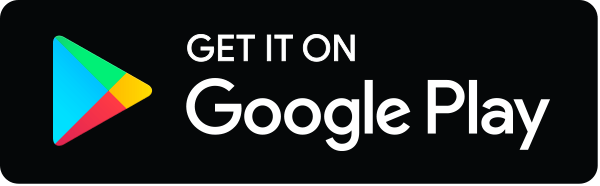
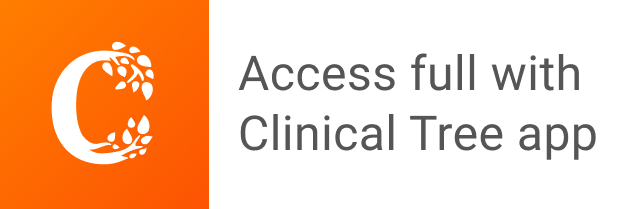