Chapter 3 Clinical and advanced techniques for optimizing MRI in refractory focal epilepsy
MRI-Negative Epilepsy, ed. Elson L. So and Philippe Ryvlin. Published by Cambridge University Press. © Cambridge University Press 2015.
Introduction
Temporal lobe epilepsy secondary to mesiotemporal sclerosis, and extratemporal lobe neocortical epilepsy secondary to dysplasias are the two most common drug-resistant epilepsies amenable to surgery. In both syndromes, early identification of these anomalies allows timely resective surgery, limits the long-term effects of recurrent seizures and medication, and has been shown to have positive consequences on cognitive outcome and brain development.
Magnetic resonance imaging (MRI) is a pivotal component in the investigation of any form of epilepsy because of its unmatched ability in visualizing brain pathology. In particular, MRI has transformed the evaluation and management of patients with drug-resistant epilepsy by allowing reliable detection of the structural lesion associated with the epileptogenic zone, thus leading to increased rates of successful resective surgery [1]. Despite technical improvements in MRI hardware and sequences, however, best-practice MRI is unremarkable, and thus unable to reveal the potential surgical target in about 50% of patients [2]. Notably, however, in many centers, despite the high efficiency in providing good EEG interpretation, MRI is still fully outsourced to the radiology department and usually includes only basic imaging. It can be argued that epileptology must include responsibility for good-quality and advanced imaging optimized for the patient’s particular problem.
It is becoming increasingly clear that both hippocampal sclerosis and neocortical dysplasias constitute a spectrum of histopathology, clinical, and radiological presentations broader than originally suspected [3–9]. Indeed, epilepsies initially considered MRI-negative are not necessarily nonlesional since in 30% to 50% of those patients who undergo surgery, histological examination of the resected specimens reveals dysplasias [2, 3], mild hippocampal sclerosis [10–12], or subtle isolated neocortical or hippocampal gliosis [13, 14]. Importantly, retrospective assessment of preoperative MRI scans, guided by quantitative structural image analysis, can frequently identify a lesion. These findings reinforce the importance of obtaining high-quality, if needed, multiple-image datasets interpreted by MRI experts to evaluate and treat patients with so-called “MRI-negative” epilepsy.
The definition of epilepsies with negative MRI is a moving target that changes with advances in diagnostic technology. While a consensus exists that the primary histopathological substrates are subtle hippocampal sclerosis and dysplasias, the MRI signature of these entities is not yet fully defined, mainly because of the lack of a unified methodology to evaluate their underlying structural changes. The purpose of this chapter is to give an overview of methods that have significantly improved the detection of subtle brain lesions in drug-resistant epilepsy. After presenting clinical protocols aimed at optimizing visualization, we will discuss advanced protocols including quantitative image acquisitions and processing methods.
Optimizing conventional structural mri
Broadly speaking, as the field strength increases, the signal-to-noise ratio (SNR) in an image increases approximately linearly. Consequently, the advent of high-field magnets at 3 Tesla, combined with the use of phased arrays instead of a conventional quadrature coil, has resulted in accelerated image acquisition, and improved signal- and contrast-to-noise ratios for a more detailed and complete characterization of structural changes in the hippocampus or the neocortex than those obtained at 1.5 Tesla. While these systems are becoming the new high-field standard, their clinical value strongly depends on the expertise of the individual who interprets the images. Importantly, evidence suggests the highest sensitivity for detecting subtle epileptogenic lesions is achieved when using epilepsy protocols that are evaluated by an experienced reader with proper clinical information [15, 16].
The ideal sequence should provide high spatial resolution, high contrast, and complete brain coverage. An increase in voxel resolution is an empirical choice based on acceptable image quality parameters and clinically reasonable acquisition time. However, there is a cost in terms of SNR that is paid for such increase. Recently commercialized 32-channel phased-array head coils provide an elegant solution to this challenge leading to significant signal-to-noise increase and obviating the use of small single surface coils. Although sensitivity of these multiple arrays is higher at the neocortex, up to 30% increase can be achieved in the mesial temporal lobes compared to an 8-channel head coil, and up to 60% with respect to a conventional quadrature coil. Consequently, a number of sequences implemented on 3 Tesla scanners currently offer superb anatomical images at submillimeter resolution (Figure 3.1).
Figure 3.1 High-resolution submillimetric MRI at 3 Tesla with 32-channel phased-array coils. Compared to the 1 mm isotropic T1-weighted images (left panels), T1- and T2-weighted images at micrometric resolution (middle and right panels) unveil anatomical details of the mesiotemporal lobe that are essential for reliable quantitative studies, such as volumetry.
In our experience, high-resolution magnetization prepared rapid acquisitions with gradient echo (MPRAGE) sequences provide excellent contrast and significantly reduced partial voluming effects; they have been documented to be superior for digital image processing. The T1-weighted inversion recovery (IR) also offers a high GM/WM contrast, which improves the visual distinction between gliotic changes and normal tissue, and the visualization of medullary veins and enlarged perivascular spaces. The T2-weighted images are useful for detecting signal changes, usually hyperintensity, associated with gliosis. As current 3 Tesla systems offer flexibility in parallel imaging performance, such as generalized autocalibrating partially parallel acquisitions (GRAPPA), it is now possible to acquire multispectral volumetric images (T1, IR, T2, and FLAIR) with isotropic resolution equal or below 1mm3 voxels in about 30 minutes, obviating the need for dedicated epilepsy protocols as the rater can manipulate and reformat the image in any plane without losing resolution. Nevertheless, when evaluating the temporal lobe, particularly mesiotemporal structures, in addition to the above-mentioned volumetric sequences, it is advisable to acquire 2D T2-weighted images perpendicular to the long axis of the hippocampus with an in-plane resolution of 0.4 to 0.5 mm3 and a slice thickness of 2 to 3 mm, which many systems allow to achieve in about 5 minutes. The practical implication of high-field scanners becoming increasingly available for clinical imaging is that patients with normal-appearing 1.5 Tesla MRI should be re-examined at 3 Tesla. Unfortunately, however, clinical radiologists may choose to evaluate lower-resolution images that have been reconstructed into thicker slabs instead of the original high-resolution volumetric acquisition as a means to decrease the number of sections to inspect. For example, T1 or FLAIR images acquired originally at 1 mm3 isotropic resolution are resampled at 3 mm, at times with the addition of interslice gaps. This procedure is obviously detrimental, as in patients with drug-resistant epilepsy the highest resolution is associated with lesser partial volume effects, thus favoring the detection of subtle anomalies (Figure 3.2).
Figure 3.2 Image resampling vs. original resolution. Axial 3 Tesla FLAIR images of a patient with histologically proven focal cortical dysplasia ILAE type IIb. The radiological evaluation was initially performed on images reconstructed from the original 3D high-resolution 1 mm acquisition into thick 3 mm slabs (upper panels), and has been reported as unremarkable. The repeated inspection of the original 1 mm isotropic images (lower panels), however, revealed a subtle dysplasia, mainly characterized by blurring of the lesional boundaries (seen on all the slices, arrows) and a minute transmantle sign (arrow head). Slice numbers for each dataset are shown.
While 7 Tesla systems appear quite promising for an even more detailed characterization of epileptogenic lesions [17], it is currently unclear if they will replace optimized 3 Tesla systems to become the new clinical standard for structural MRI. Challenging disadvantages of ultra-high field imaging include far greater radiofrequency signal inhomogeneities, higher energy deposition in tissue, as quantified by specific absorption rate (SAR), and more pronounced imaging artifacts from static field inhomogeneities at soft tissue–air and soft tissue–bone interfaces. To remain within the SAR limits, the number of slices per measurement often has to be decreased, especially in spin-echo T2- and FLAIR-weighted sequences. Thus, coverage of the entire brain at high in-plane resolutions cannot currently be achieved at 7 Tesla within a single spin-echo sequence. With appropriate coils, pulse sequence modifications, and imaging protocol optimizations, however, it is likely that 7 Tesla scanners will be used without losing key information obtained at lower field strengths.
Image processing of structural mri
In many patients, routine visual MRI inspection does not permit diagnosis of epileptogenic lesions with a sufficient degree of confidence or it is simply unremarkable. This clinical difficulty has motivated the development of computer-aided methods aimed at analyzing brain morphology and signal intensities. These procedures provide distinct information through quantitative assessment without the cost of additional scanning time or exposure to ionizing radiation. Whereas the general tendency is to automate analyses generating results that are both replicable and rater independent, it is advisable that the fundamental image preprocessing steps (e.g., intensity nonuniformities correction, registration, and tissue segmentation) remain accessible to the user for purposes of quality control.
Temporal lobe epilepsy
Hippocampal sclerosis is the histopathological term used to describe neuronal loss and astrogliosis in the hippocampus proper, particularly CA1 and CA4, and the dentate gyrus [4, 18]. Although these features are commonly examined at the level of the midbody of the hippocampus, there is significant variability in their extent and severity. In addition, as tissue obtained from surgical resections may be incomplete due to partial resections or fragmented as a result of subpial aspiration, a comprehensive evaluation of the mesiotemporal lobe structures is limited; a neuropathological assessment of the entire hippocampus, the amygdala, and entorhinal cortex in the same patients is thus rarely done. Nevertheless, when available, surgical specimens and postmortem data have shown evidence for extended pathology involving also the entorhinal cortex and amygdala [19]. Human pathological data on neocortical abnormalities outside the temporal lobes has also been infrequent due to difficulties in obtaining postmortem specimens. In their seminal study, Margerison and Corsellis [20] described neuronal loss and gliosis in frontal and occipital cortices in 22% of patients. In a single patient who underwent temporal lobe surgery, another autopsy report showed varying degrees of architectural abnormalities involving virtually all lobes [21].
Quantitative analysis of the mesiotemporal lobe
For the last two decades, MRI volumetry has been the most commonly employed quantitative technique to assess mesiotemporal lobe pathology as it is more sensitive than visual evaluation. In TLE, hippocampal atrophy is considered a surrogate marker of hippocampal sclerosis; the degree of atrophy correlates with the severity of neuronal loss in the cornu ammonis, particularly CA1 [22]. The utility of hippocampal volumetry stems from its ability to lateralize the seizure focus in about 70% of cases at the individual patient level [23]. Yet, in clinical settings, manual volumetry of mesiotemporal lobe structures, often restricted to the hippocampus, is largely underutilized as it is considered prohibitively time consuming and requires an anatomy expert to perform the task. Automatic hippocampal segmentation studies in TLE have been sparse and many have provided up until now rather unsatisfactory results [24–26]. We developed a robust and reliable algorithm that integrates deformable parametric surfaces and multiple templates in a unified framework [27]. Our method showed excellent overlap with manual labels, achieving submillimetric accuracy in patients, regardless of the degree of atrophy, that was virtually identical to that obtained in healthy controls. Importantly, the produced labels of the various structures may be used for more advanced processing, as detailed below.
Since volumetry provides a global estimate of atrophy, its sensitivity to detect subtle diffuse or focal anomalies is limited. This may explain why in 30–40% of patients with unambiguous electroclinical features of drug-resistant TLE, hippocampal volumetry is unremarkable even though histopathology reveals subtle sclerosis. Indeed, surgical specimens in MRI-negative TLE have clearly demonstrated 20% cell loss in the hippocampal CA1 subfield [4, 12] or isolated gliosis. Neuronal loss may predominate in the entorhinal cortex or the amygdala [19]. In light of these observations, entorhinal cortex volumetry provides a valuable alternative, lateralizing the focus in 25% of cases with normal hippocampal volume [11]. Shape analysis has the potential to further refine the MRI correlates of hippocampal pathology [28]. We have developed and validated a 3D surface-based method relying on spherical harmonic shape descriptors that localizes submillimetric variations of volume between a given structure and a template while guaranteeing anatomical correspondence across subjects [29], a requisite for reliable statistics. In our experience, this technique is effective in detecting subtle atrophy in patients with normal whole hippocampal volume (Figure 3.3).
Figure 3.3 Hippocampal shape analysis. Coronal 3 Tesla T1- and T2-weighted MRI and hippocampal surface maps of atrophy normalized with respect to healthy controls (z-score, shown by the color bar) for two patients with right TLE. In the first patient (A), the right hippocampus is clearly atrophic and shows T1 hypo- and T2 hyperintensity. Surface-based analysis confirms and localizes areas of atrophy along the rostrocaudal extent of the right hippocampus. In case (B), the inspection of conventional MRI was reported as normal. Conversely, the surface-based maps reveal subtle atrophy, at the level of the right hippocampal head and the body. Histology confirmed the presence of subtle hippocampal sclerosis. Dotted lines on the surface maps indicate the level of the coronal MRI cuts. Right is right on the panels.
Compared to the visual analysis of T2-weighted MRI, T2-relaxometry, which provides a quantitative estimate of T2-weighted signal, has been shown to yield an increased sensitivity in detecting mesiotemporal gliosis [30–32]. We demonstrated that hippocampal T2-relaxometry correctly lateralizes the seizure focus in 82% of patients with normal hippocampal volume [31]. We also showed that prolongation of white matter T2 signal in the temporal stem occurs in about 70% of these patients; in half of them the increase was bilateral and symmetric. However, white matter T2-relaxometry provided a correct lateralization of the seizure focus in a third of patients, demonstrating that such measurement may provide complementary information [32].
Quantitative analysis of the neocortex
Since the early 2000s, the technique of voxel-based morphometry (VBM) has been extensively used to assess structural abnormalities in TLE as it permits an automated whole-brain structural analysis without prior anatomical segmentation. However, for detecting focal pathology within the mesiotemporal lobe, voxel-based measurements are insufficiently sensitive, especially when pathomorphological changes are relatively subtle, as is the case in hippocampal sclerosis [33]. On the other hand, VBM [34] and measurement of cortical thickness [35, 36] offer a sensitive means for assessing whole-brain structural integrity. In drug-resistant TLE, they have shown widespread atrophy and evidence for disease progression, particularly in the frontocentral areas [37]. Notably, we demonstrated that patients with obvious hippocampal atrophy and those with normal hippocampal volume have similar patterns of static and dynamic pathology in neocortical regions remote from the seizure focus [35]. Equivalent neocortical dynamics occurring despite different degrees of mesiotemporal lobe pathology supports the concept that these two TLE entities are part of the same spectrum and that longitudinal changes are most likely reflective of secondary effects of seizures. In light of functional data showing progressive cognitive decline and extension of the epileptogenic network in relation to recurrent epileptic discharges, these results provide compelling evidence that drug-resistant TLE, regardless of the degree of hippocampal atrophy, is a progressive disorder that warrants early surgery.
The majority of studies dedicated to the assessment of the neocortex have been group based. Preliminary data suggest, however, that machine-learning techniques applied to whole-brain automated segmentation may aid lateralizing the presumed seizure focus in single subjects with visually normal MRI [38]. Nevertheless, as features selected by the classifier may challenge biologically plausible interpretations, the clinical use of these techniques warrants cross-validation through independent studies.
Focal cortical dysplasia
Neocortical epilepsy related to focal cortical dysplasia (FCD) accounts for more than half of pediatric and a quarter of adult patients [3]. Main FCD features on structural MRI include abnormally thick cortical gray matter (50–92% of cases) and blurring of the gray–white matter interface (60–80% of cases) [3, 39]. Analysis of T2-weighted images reveals gray matter hyperintensity in 46–92% of lesions and sensitivity of FLAIR images is even higher (71–100%). The typical transmantle sign, a footprint of disrupted cell migration along radial glial processes, presents as a funnel-shaped hyperintensity extending from the ventricle to the lesion and is seen in the majority of FCD type II cases [7, 39, 40].
The in vivo visibility of dysplastic changes on MRI generally parallels the degree of histopathological derangement [3]. Even in patients with FCD type II, however, as the radiological spectrum on MRI encompasses variable degrees and patterns of gray and white matter changes, visual identification can be challenging, particularly when inspecting the convoluted neocortex in two-dimensional images. Indeed, recent surgical series indicate that up to 33% FCD type II and 87% of FCD type I [7, 8, 39] present with unremarkable routine MRI, underlining the limited power of conventional imaging to resolve subtle cortical dysplasia.
Gyral anomalies may be the only visible MRI sign of cortical dysgenesis. Using automated sulcogyral morphometry, we demonstrated that 85% of small FCD lesions, primarily those overlooked during conventional radiological inspection, are located at the bottom of an abnormally deep sulcus [41]. Importantly, such evidence can guide the search for migrational anomalies in patients in whom large-scale MRI features are only mildly abnormal or absent.
Several groups have applied VBM to detect structural abnormalities related to MRI-visible FCD in single patients [16]. This fully automated image processing method, which identifies differences in tissue density at a voxel level, detects increases in gray matter concentration colocalizing with the lesion in 63–86% of cases. Histopathological confirmation of lesions that eluded visual inspection (despite their relatively large size) [42] suggests that VBM may be applied to investigate patients with MRI-negative epilepsy. Importantly, however, a threshold of 2SDs above the mean gray matter concentration in healthy controls does not guarantee specificity of findings since, at this threshold, false positives may occur in control subjects. Voxel-based comparison has also been used to analyze intensities derived from quantitative MRI contrasts such as T2-relaxometry, double inversion recovery, and magnetization transfer imaging. These approaches have shown high sensitivity (87–100%) in detecting obvious malformations of cortical development [43–45]. Nevertheless, these techniques may identify areas concordant with clinical and EEG findings in less than a third of MRI-negative cases and have low specificity [44, 46].
The relatively unspecific nature of VBM with respect to pathological characteristics of FCD has motivated the search for computer-based models of morphological imaging features distinctive of dysplasias. Using models of cortical thickness, blurring, and tissue intensity derived from 3 T1-weighted images [47] and combined into a single composite map, the sensitivity of visual identification of histologically proven FCD may be increased up to 40% relative to conventional MRI, while maintaining high specificity [48]. Furthermore, blending these models with the quantification of high-order image texture features that cannot be discriminated by the human eye, we were able to detect automatically about 80% of dysplastic anomalies [49, 50]. One limitation of voxel-based approaches is that they do not fully take into account the complex topology and are therefore prone to volume averaging of nonadjacent cortical regions across sulci, potentially increasing the false-positive rates. In our experience, a surface-based approach preserving topographic anatomy is effective in detecting small lesions (Figure 3.4).
Figure 3.4 Surface-based computational models of focal cortical dysplasia. In this patient with frontal lobe epilepsy, high-resolution 3 Tesla MRI was reported as normal, likely because of the subtle nature of the dysplasia, as demonstrated by the coronal T1-weighted MRI section (right lower corner). The upper panels display the T1-weighted derived computational models normalized with respect to controls (z-score). The cortical thickness map is unremarkable. Conversely, the gray matter intensity map and the gradient map (modeling gray–white matter blurring) show significant anomalies. The composite map identifies the lesional area as the only anomaly compared to controls. Histopathology of the surgical specimen confirmed the presence of focal cortical dysplasia ILAE type IIb.
We have adopted this framework also for fully automated lesion detection based on neural networks that successfully identified 89% of small FCD lesions overlooked by experts [41].
Overall, the most relevant clinical impact of post-processing is that cases considered MRI negative at first have been increasingly recognized as secondary to FCD type II, particularly FCD type IIa.
Diffusion tensor imaging
Diffusion tensor imaging (DTI) makes inferences about the integrity of axon and myelin sheaths through the analysis of passive water diffusion relative to white matter tracts [51]. In TLE, fractional anisotropy (an index of deviation of water diffusion from a random spherical displacement) is consistently decreased in temporolimbic tracts [52–54]. Nevertheless, the ability of DTI to lateralize the focus has been disappointing [55]. Notably, the conventional whole-tract approach yielding a single value per diffusion parameter per tract reduces sensitivity for the detection of subtle focal changes. Relative to the widespread pattern of anisotropy changes, mean diffusivity anomalies (a marker of bulk diffusion) follow a more restricted distribution [52]. To overcome this limitation, we measured the spatial distribution of diffusion indices along tracts carrying temporal lobe connections and showed that the effect size of diffusivity alterations decreases as a function of anatomical distance to the temporal lobe, suggesting colocalization with the focus [56]. Importantly, our segmental analysis allowed us to correctly lateralize 100% of MRI-negative TLE patients (i.e., with normal hippocampal volumes). On the other hand, whole-tract analysis identified the epileptogenic hemisphere in only 85% of cases.
In patients with MRI-visible FCD, regions of interest [57] and whole-brain voxel-based analyses [58] have shown abnormalities in diffusion indices in the subcortical white matter adjacent to the malformation. Nevertheless, in some patients with no visible MRI anomaly, focal changes may colocalize with the EEG focus [58–60] (Figure 3.5).
Figure 3.5 Surface-based diffusion tensor imaging in focal cortical dysplasia. The upper panels show the outline (in yellow) of the gray matter portion of the lesion on the apparent diffusion coefficient (ADC) map, and on the T1- and T2-weighted MRI. Lower panels show the mean ADC map of the immediate subcortical white matter in ten healthy controls. In the patient, both the mean and z-score ADC maps show abnormally increased values in the white matter below the FCD lesion. These changes indicate microstructural abnormalities extending beyond the visible lesion.
Yet, abnormalities often extend beyond the epileptogenic region and the visible FCD [61], highlighting the need to balance sensitivity and specificity. Moreover, the underlying nature of diffusion abnormalities needs to be clarified, as correlative studies have been so far limited to a single patient [62].
Magnetic resonance spectroscopy
Magnetic resonance spectroscopy (MRS) measures in vivo the concentration of metabolites, including N-acetylaspartate (NAA), choline (Ch) and creatine (Cr). Spatially selective excitation and refocusing pulses allow acquiring a single volume (voxel), typically 1 to 8 cc. Alternatively, spectroscopic imaging allows collecting data from multiple locations simultaneously. Numerous factors contribute to the advantages of performing spectroscopy at 3 Tesla. Primarily, the stronger chemical shift at higher fields increases the spread of individual peaks, resulting in improved spectral resolution and identification of metabolites that were obscured at 1.5 Tesla, such as GABA and glutamate. Also, the improved SNR increases the signal derived from each metabolite, so that their peaks are easier to differentiate from background noise.
Decreases in NAA, a compound specific to neurons, may lateralize the seizure focus [23] and predict surgical outcome [63] in drug-resistant TLE, thus yielding potential clinical value. Seizure focus lateralization in patients with no visible hippocampal sclerosis, however, has been disappointing [64]. Moreover, patients with neocortical epilepsy often have poorly defined epileptogenic regions, posing significant additional demands with regard to the selection of the brain region to explore, and the type of data acquisition (single voxel versus chemical spectroscopic imaging). Nevertheless, in frontal lobe epilepsy, MRS has provided some encouraging results [65, 66]. Using proton spectroscopy, it is also possible to measure both in vivo GABA and glutamate, the primary excitatory neurotransmitter in human brain. Increases in glutamate, have been observed in association with the ipsilateral hippocampus in MRI-negative TLE [67, 68]. Due to low signal to noise, however, attempts to measure GABA within the putative seizure focus have produced mixed results [69, 70].
Overall, spectroscopy in MRI-negative epilepsies should be regarded as a complimentary investigation.
Conclusion
By revealing subtle lesions that previously eluded visual inspection, quantitative image analysis, particularly image processing of structural MRI, has clearly demonstrated increased sensitivity compared to conventional techniques. Importantly, as advanced image post-processing can be performed on clinical MRI yielding 3D millimetric or submillimetric multicontrast images, they offer a substantial cost–benefit. Based on our experience and mounting evidence from the literature, we propose the term MRI-negative be used in patients in whom both the visual inspection and quantitative image processing analyses at 3 Tesla interpreted by an epileptologist with expertise in neuroimaging are unremarkable.
Importantly, to date, the MRI signature of FCD type I remain imprecise. Nevertheless, as lesions in patients with MRI considered nondiagnostic may possibly differ only slightly from normal tissue and present unanticipated traits, future methods applied to these cohorts should entail the design of statistical models of morphology and signal in a multivariate framework rather than be based on the current unimodal approaches. Such efforts, ideally refined through correlative histopathology, will help clinicians devising better criteria to perform timely interventions that achieve seizure control, turning these challenges into opportunities for better patient care.
References












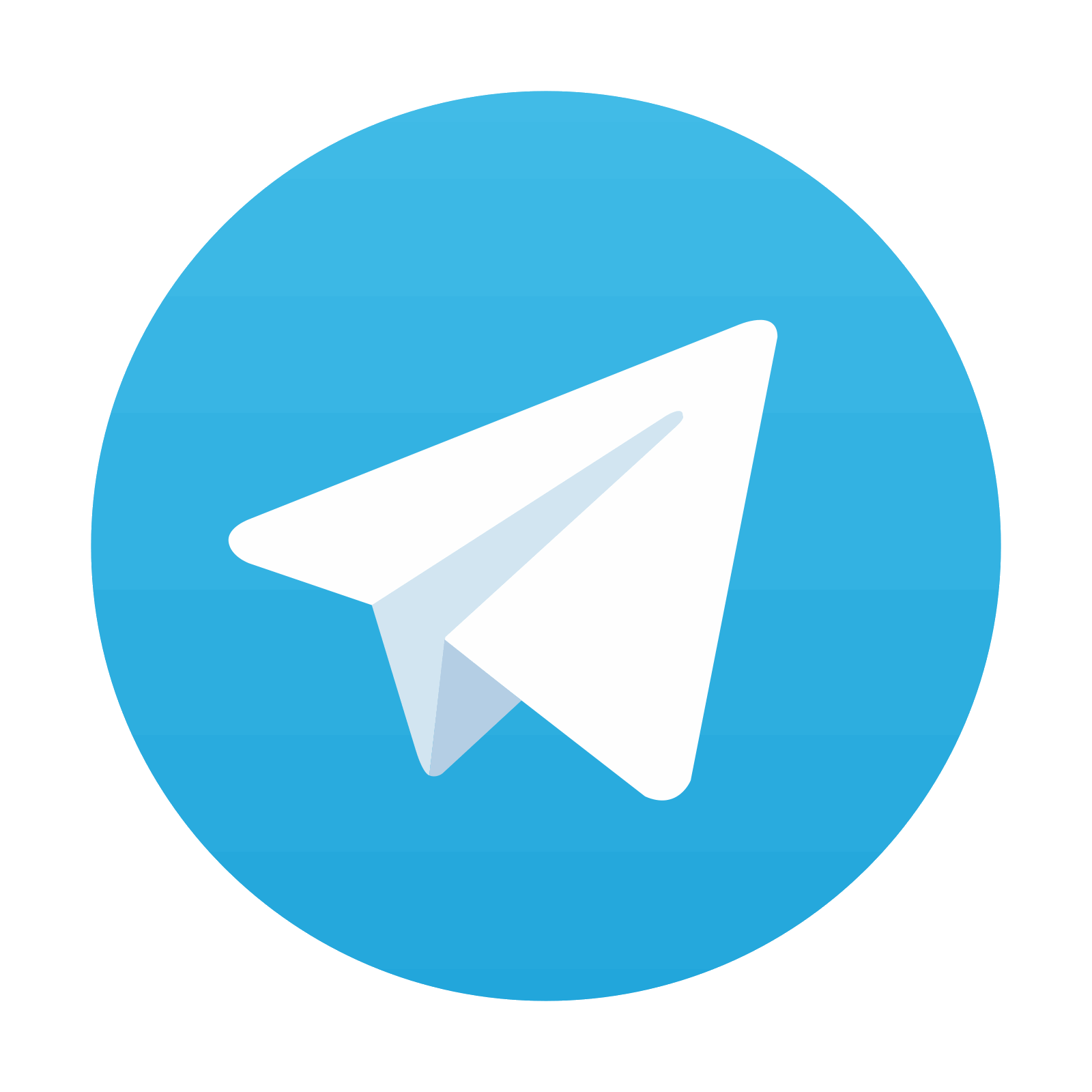
Stay updated, free articles. Join our Telegram channel
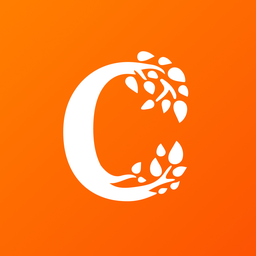
Full access? Get Clinical Tree
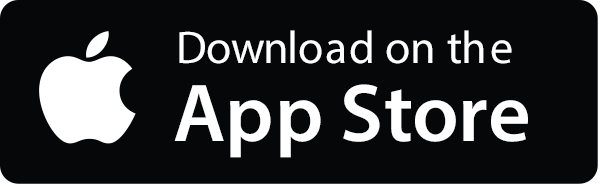
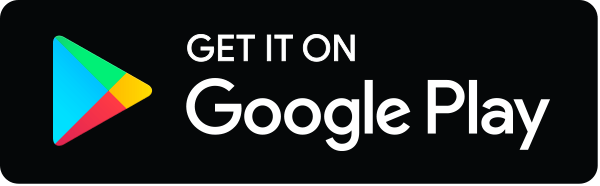
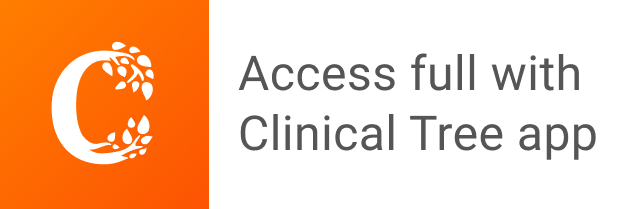