Abstract
Severe traumatic brain injury (TBI) includes a heterogenous set of injury patterns and affects a wide range of population groups including children, young adults and the elderly. It can cause death or disabling morbidity and is associated with significant socioeconomic burden.
Following the primary injury, a series of metabolic, inflammatory and vascular processes occur which may lead to secondary tissue damage. Additionally, patients with severe TBI are also at increased risk of systemic complications.
The initial resuscitation and subsequent critical care management of patients with TBI focuses on limiting secondary brain injury. This requires a systematic approach to avoid physiological disturbances which may worsen cerebral oxygen delivery and energy substrate metabolism. Factors such as hypotension, hypoxaemia, hypo- and hyperglycaemia and hyperthermia can directly exacerbate the secondary injury and lead to worse outcomes. The maintenance of cerebral perfusion with adequate cerebral oxygenation and energy substrate delivery is critical but may be challenging to achieve.
Management protocols have evolved with international consensus, providing guidelines that can assist clinicians in delivering optimal care.
Introduction
Brain trauma includes a heterogenous group of injury patterns followed by complex, multisystem pathophysiological responses. Young adults, typically injured by high energy forces, often have concurrent extra-cranial injuries while older adults, injured following relatively low energy mechanisms, are more likely to be affected by comorbidities at the time of injury. The heterogeneity of traumatic brain injury (TBI) limits the generalisability of trial data, and the nuances of TBI management may require a knowledge-based approach rather than an evidence-based approach. A clear understanding of the pathological processes is therefore essential to providing high quality neurocritical care. Although brain trauma produces a diverse range of injuries, the Glasgow Coma Scale (GCS) can been used to stratify mild, moderate and severe TBI. A post-resuscitation GCS of 8 or less represents severe TBI. Severe TBI is typically managed in a neurosciences intensive care unit.
Pathology
The brain is usually protected from mechanical forces by the bones of the skull, the meninges and a layer of cerebrospinal fluid (CSF). However, excessive force can overwhelm these mechanisms to produce a range of focal and diffuse injury patterns. While the strength and rigidity of the skull are critical for reducing primary injury to the brain, there is a biological cost associated with mechanically protecting the brain in this way: the skull and the reflections of the dura are rigid structures and the brain is therefore enclosed within a number of non-compliant compartments. An increase in volume within any compartment, caused by haematoma or oedema, for example, is tolerated poorly. The expanding mass displaces venous blood and CSF, but when this capacity is exhausted, further increases in the intracranial volume will lead to a rapid increase in intracranial pressure (ICP). A global increase in ICP can impair cerebral perfusion while raised pressure within a localised area of brain can create a pressure gradient, which will cause shearing injury or herniation from one compartment to another.
The site and mechanism of injury has an important effect on the pattern of tissue damage, treatment options and prognosis. A direct blow to the head generally causes cerebral contusions and intracranial haemorrhage. Contusions occur when microhaemorrhages form as a consequence of damage to small blood vessels. Intra-axial haemorrhage, within the brain parenchyma, causes direct neuronal injury, but even extra-axial haemorrhage (e.g. subdural or extradural haemorrhage) can cause significant injury as a result of the mass effect.
Vehicle accidents and violent assault can cause rapid acceleration or deceleration forces which cause shearing at the interface between tissues of different density. This typically affects the grey matter/white matter junction and causes immediate axonal injury.
Penetrating injuries cause a spectrum of laceration, contusion and haemorrhage but are additionally complicated by disruption of the meninges and the introduction of foreign material with potentially infectious sequelae.
Secondary injury occurs in response to the primary event but develops beyond the injury site. Following the primary injury, a series of metabolic, inflammatory and vascular processes occur, which produce tissue damage through direct intracranial lesions, such as cerebral oedema, or haemorrhage extension. There are a number of cellular processes that lead to secondary brain injury. Excitotoxicity occurs as cell injury causes postsynaptic membranes to depolarise, leading to an uncontrolled influx of calcium ions and the release of excitatory neurotransmitters. High intracellular concentrations of calcium also activate enzymatic processes and ultimately lead to cellular death. Cellular damage also causes increased cell membrane permeability and water influx leading to cytotoxic oedema or cell rupture. Vasogenic oedema occurs as disruption of the endothelium leads to the uncontrolled transit of electrolytes and water into the extra-cellular space.
Additionally, patients with severe TBI are at risk of systemic complications, and neuronal death may occur as a result of systemic causes (e.g. hypotension, hypoxaemia or hypoglycaemia). The brain is particularly vulnerable to secondary injury due to its high metabolic demands. The oxygen requirement in the cortical grey matter is approximately 5 ml 100 ml−1 min−1, and this is usually met by very high blood flow (about 20% of cardiac output). Brain injury disrupts this balance and the supply of energy substrate falls while demand is increased. There are also complex pathophysiological changes to glucose uptake which can lead to metabolic crisis even in the presence of adequate blood flow. It is therefore important to avoid seizures and hyperthermia which increase cerebral metabolic demands, and oxygen and glucose delivery should be maintained by avoiding hypoxia, anaemia and hypoglycaemia.
Following traumatic injury, neurones and glial cells release pro-inflammatory cytokines which produce both a local and a systemic inflammatory response. Disruption of the blood–brain barrier allows these inflammatory mediators to leak into the circulation and a systemic inflammatory response is sustained. Disruption of the autonomic and neuroendocrine pathways can also cause immunosuppression, which is associated with a high rate of infective complications, such as pneumonia, and occurs commonly in TBI patients.
Immediate Management
A combination of primary and secondary injury processes will contribute to neurological damage; however, only the secondary injury is amenable to intervention and is therefore the focus of almost all current therapeutic options.
Modern trauma systems allow critical care management of TBI to be initiated rapidly by pre-hospital teams. The capabilities of the responder and the available resources may vary, but the clinical priorities remain the same. A rapid assessment needs to be performed, usually in parallel with the initial resuscitation. Life-threatening injuries need to be identified and treated, and the patient must then be transported to a trauma centre.
A number of pre-hospital interventions can reduce secondary brain injury. Tracheal intubation may be performed to control hypoxia or ventilation and to facilitate the transfer of agitated and combative patients. Haemorrhage control and the administration of fluids (including blood and blood products) can improve cerebral oxygen delivery and osmotherapy can be used to rapidly improve cerebral perfusion.
While these interventions are valuable, scene times should be minimised and urgent transfer to appropriate definitive care is essential. Despite high quality, rapid intervention many patients with severe TBI will have already sustained a secondary injury by the time they arrive in hospital. Hypotension, normally as a result of extra-cranial injuries, and hypoxia, as a result of aspiration or airway obstruction, frequently co-exist in the early stages of TBI and have been shown to be associated with increased mortality.
When the potentially brain injured patient arrives in the Emergency Department a rapid assessment needs to be made to ensure that any immediate life-threatening conditions are identified and treated. The first priority is usually to ensure the airway is patent; this may require tracheal intubation. The pharyngeal and laryngeal reflexes are frequently impaired in patients with severe TBI, and it may also be necessary to intubate the trachea to prevent aspiration of gastric contents. If the decision is made to intubate, it is important to use an appropriate technique that avoids precipitating hypotension, hypoxia or a significant increase in ICP. While intubation may be an emergency in the context of airway obstruction, there is often time to perform a focused neurological examination to determine the GCS, pupillary size and reactivity, and the presence of signs of spinal cord injury. Following the induction of anaesthesia, further clinical assessment will be limited. Cervical spine injuries need to be considered in conjunction with airway management, some injuries may be potentially unstable and there is a risk of iatrogenic spinal cord injury.1 There is some controversy about cervical spine immobilisation, but it is important to consider the risk of cord injury particularly during intubation. Manual inline stabilisation (MILS) should be used during laryngoscopy and the use of more advanced airway techniques, such as video laryngoscopy, may help to limit the forces transmitted to the cervical spine.
Despite a general consensus on the early management goals in TBI treatment, there is little empirical evidence to support specific physiological targets, and consequently there is controversy regarding specific resuscitation endpoints. Periods of hypotension should be strenuously avoided: the current Brain Trauma Foundation guidelines recommend an initial systolic blood pressure target between 100 and 110 mmHg to maintain cerebral perfusion. Other early resuscitation priorities include maintaining normothermia and glycaemic control.
Early computed tomography (CT) imaging is necessary to identify the type and severity of the injury and resuscitation may occur in parallel with preparation for imaging.
There are very few pharmacological agents that have been proven to be beneficial in early severe TBI. A meta-analysis of randomised controlled trials of tranexamic acid in TBI suggests a reduction in haemorrhage extension and mortality; however, the data were inconsistent. The CRASH-3 trial2 evaluated the safety and effectiveness of tranexamic acid in isolated TBI and demonstrated that early administration was associated with a reduction in the risk of death in moderate and mild head injury; however, there was no survival benefit in the severe TBI group.
Patients who have been taking anticoagulants prior to the injury and patients who are coagulopathic should have their clotting corrected to avoid haemorrhage extension. Patients who are treated with anti-platelet drugs prior to injury have worse outcomes following TBI; however, correcting platelet inhibition with platelet transfusions is not associated with improved outcomes.3
Critical Care Management
High quality critical care management is essential and most patients with severe TBI are cared for in a regional neuroscience intensive care unit (NICU). These specialist centres provide advanced monitoring, imaging and neurosurgical input, and they are typically associated with better outcomes.4 However, simple measures are also important, and elevating the head of bed at 30–45°, for example, can significantly reduce ICP and improve cerebral perfusion.
Assessment
Neurological examination is an important assessment tool, even for patients who are unconscious or sedated. Deterioration may be identified by the regular assessment of the GCS and measurement of pupil diameter and reactivity to light. However, there are many factors that can make this basic assessment difficult. The motor response is the most consistently assessable component of the GCS score, and a GCS assessment may be inaccurate if tracheal intubation prevents a verbal response or facial injuries prevent eye opening.
Pupillary diameter and reactivity are important clinical signs but can be affected by a variety of mechanisms including oculomotor or optic nerve injury, localised eye injuries, and both pontine and midbrain dysfunction. The underlying injury should also be considered: an isolated unilateral third nerve palsy will not affect the consensual light reflex, while an optic nerve injury will disrupt both direct and indirect responses. Loss of the pupillary reflex is usually an indicator of more severe injury and 70–90% of patients with bilaterally unreactive pupils will have a very poor outcome.
Pupillary reaction to light can be assessed using a torch, although this is associated with poor inter-rater accuracy. Automated pupillometry uses a handheld device to measure pupil size and light reactivity with a high degree of accuracy and is especially valuable when the pupil is small.
Despite the value of bedside clinical assessment, neuroimaging is essential for diagnosis, to guide surgical interventions and for prognosis. Access to early imaging is associated with improved outcomes and fewer life-threatening complications. CT is the most commonly used imaging modality for guiding the care of TBI patients. However, normal CT appearances do not exclude a significant injury and injury patterns such as traumatic axonal injury (TAI) can be difficult to identify on CT. There may be a role for magnetic resonance imaging (MRI) in this setting; diffusion-weighted MRI can identify the microvascular shearing and microhaemorrhages associated with axonal injury. However, its use in the acute setting is limited because unstable patents with high ICP cannot tolerate the prolonged supine positioning required for MRI.
Systemic Management
Haemodynamic instability is frequently seen in patients with TBI and even brief periods of hypotension are associated with increased mortality. It is important to achieve adequate systemic blood pressure (SBP) so that the cerebral perfusion pressure is maintained. Fluid restriction is not recommended, and cardiac output monitoring should be used to adjust fluid administration to maintain euvolaemia. Vasopressors or inotropes may be required; however, catecholamines released following brain injury can induce myocardial ischaemia and impaired ventricular function, which may make it difficult to achieve SBP targets.
TBI can also cause disruption of inhibitory cardiovascular centres in the brainstem which can lead to the phenomenon of paroxysmal sympathetic hyperactivity (PSH). This causes cycles of agitation and dystonia with pyrexia, tachycardia, hypertension and diaphoresis. Treatment options include opiates, benzodiazepines and beta adrenergic antagonists.
Table 4.1 Physiological targets for the management of severe traumatic brain injury
Ventilation | PaO2 10–12 kPa PaCO2 4.5–5.0 kPa PEEP 5–12 mmHg VT <8 ml kg−1 (if neuroprotective approach allows) |
Cardiovascular | SBP 110 mmHg (100 mmHg if aged 50–69 years) MAP >90 mmHg Euvolaemia Haemoglobin 100 g l−1 Vaspressors / inotropes as required |
ICP and CPP | ICP <22 mmHg CPP 50–70 mmHg |
Biochemical | Normoglycaemia Serum sodium 145–155mmol l−1 |
Other | Normothermia Seizure control |
Patients with severe TBI usually require tracheal intubation and mechanical ventilation to protect the airway and to ensure adequate oxygen delivery and carbon dioxide clearance. Hypoxia can lead to secondary injury in the brain but hyperoxia can also be harmful; oxygen should be therefore be titrated to achieve a narrow range and a target arterial partial pressure of oxygen (PaO2) of 10–12 kPa is usually maintained. Managing carbon dioxide clearance is often more complex; cerebral vascular tone is dependent on the partial pressure of carbon dioxide (pCO2), and control of ventilation is therefore critically important. Hypoventilation leading to hypocapnia will induce cerebral vasoconstriction, which can result in ischaemia, while hypercapnia leads to cerebral vasodilation, an increase in intracranial blood volume and subsequent increases in the ICP. Mechanical ventilation is adjusted to achieve an optimum balance and usually a target arterial partial pressure of carbon dioxide (PaCO2) range of 4.5–5.0 kPa is maintained during the acute phase of severe TBI. Hyperventilation may be used to rapidly and temporarily control acute spikes in ICP, but this should be reserved for patients at risk of imminent life-threatening complications, such as herniation of the cerebellar tonsils into the foramen magnum.
Mechanical ventilation in TBI can be challenging and a balanced approach is often required. Catecholamines released following TBI may cause neurogenic pulmonary oedema, and the high tidal volumes required for optimum PaCO2 control may precipitate acute lung injury (ALI). Additionally, patients with TBI may have coexisting chest injuries and are at high risk of other pulmonary complications, such as pneumonia.
Patients with severe TBI are also at risk of gastrointestinal complications. Reduced gut motility is common, but increases in intra-abdominal pressure may lead to increased ICP and regular laxatives are important to avoid constipation. Stress ulceration is also common and early enteral feeding is recommended. Ulcer prophylaxis with a proton-pump inhibitor or H2 antagonist is used until enteral feeding is successfully established.
Haematological, Metabolic and Endocrine Management
Acute haemorrhage in isolated TBI rarely causes significant anaemia at presentation. However, 50% of patients with severe TBI will become anaemic during the first week.5 Anaemia is associated with worse clinical outcomes, but red cell transfusion does not appear to effectively mitigate that risk.6 The optimum haemoglobin concentration for cerebral oxygen delivery requires a favourable balance between oxygen-carrying capacity and blood viscosity. A haemoglobin target of 100 g L−1 is likely to achieve optimum cerebral oxygen delivery but achieving that target through allogenic red cell transfusion is associated with risks. Few clinical studies examining the optimal transfusion strategy in TBI have been performed and are often limited by significant confounders.7 A restrictive transfusion policy is usually implemented in general critical care patients and is likely to be safe for patients who are alert and can be reliably assessed. However, an individualised transfusion strategy is required in patients with moderate and severe TBI and a haemoglobin target of 90–100 g L−1 is usually more appropriate.
Disordered coagulation is common following TBI and both hypocoagulable and hypercoagulable states can occur. Functional assays, such as thromboelastometry, may therefore be more useful than routine laboratory tests in defining clotting abnormalities. The risk of deep venous thrombus is high, and thromboprophylaxis should be initiated early; pharmacological thromboprophylaxis is usually safe after 24 hours of clinical stability if there are no concerning signs on repeat imaging. If thromboprophylaxis is contraindicated by other injuries or an ongoing bleeding risk, then the placement of a temporary inferior vena cava filter should be considered.
Metabolic and electrolyte abnormalities are very common after TBI. Hyperglycaemia often occurs and may result in secondary brain injury. While hyperglycaemia is associated with poor neurological outcomes in TBI, intensive glycaemic control may also be harmful and therefore intermediate glucose concentrations (7.8–10.0 mmol L−1) should be targeted.8 The administration of hypotonic glucose solutions can worsen cerebral oedema and should be avoided.
Serum electrolyte disturbance, particularly dysnatraemia, is frequently seen in patients with TBI. Hyponatraemia is especially harmful because cerebral oedema is exacerbated and the seizure threshold is reduced. Hyponatraemia is usually a consequence of either cerebral salt wasting (CSW) or the syndrome of inappropriate antidiuretic hormone (SIADH). Brain injury can also disrupt the production of vasopressin and cause hypernatraemia secondary to central diabetes insipidus. Iatrogenic hypernatraemia, as a consequence of hypertonic saline solutions is common, but should be corrected gradually as an acute fall in osmolality will worsen cerebral oedema. Hypophosphataemia and hypomagnesaemia are also common and should be corrected to avoid lowering the seizure threshold.
The hypothalamic–pituitary–adrenal axis is often disrupted by brain injury, particularly by TAI, severe cerebral oedema and skull base fractures, or in older patients. Endocrine abnormalities are therefore common and secondary adrenal insufficiency occurs in many patients with severe TBI.9 Screening for pituitary insufficiency should be considered in high risk patients or those with ongoing hyponatraemia, hypoglycaemia or a persisting requirement for vasopressors. Hydrocortisone may be necessary for refractory hypotension, and fludrocortisone may be added if hyponatraemia persists. The endocrine sequelae of TBI can be protracted and may impact on the chronic phases of rehabilitation.
Table 4.2 Diagnosis and management sodium disturbances in traumatic brain injury
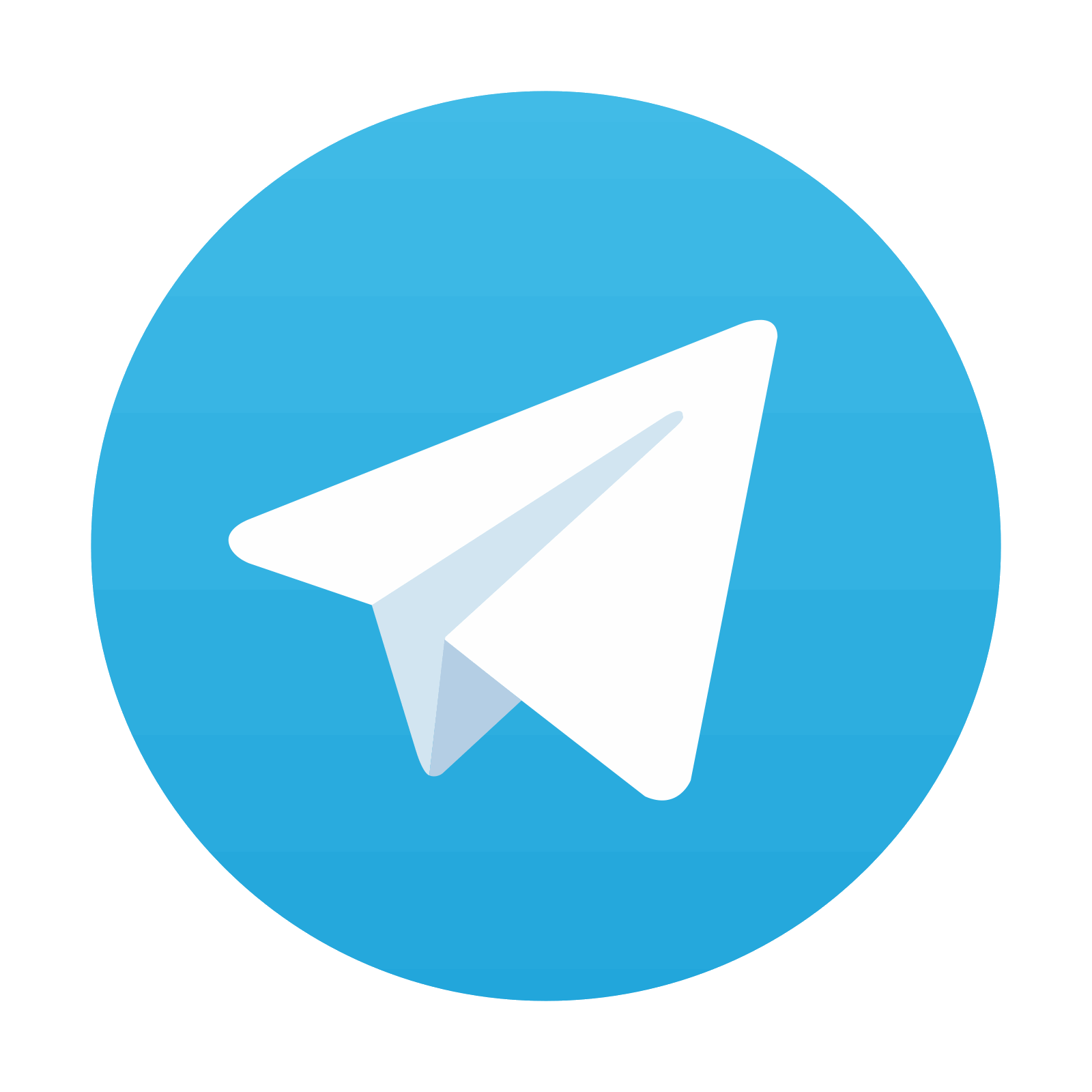
Stay updated, free articles. Join our Telegram channel
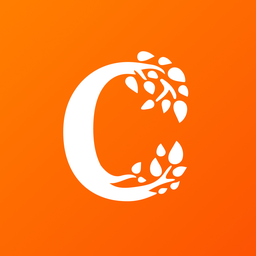
Full access? Get Clinical Tree
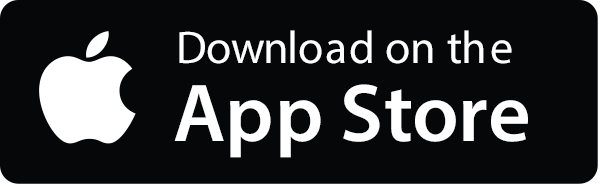
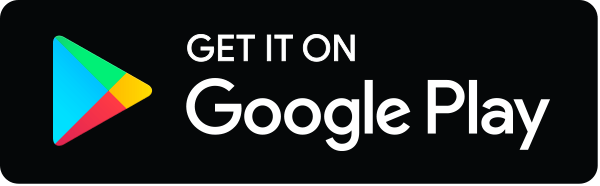
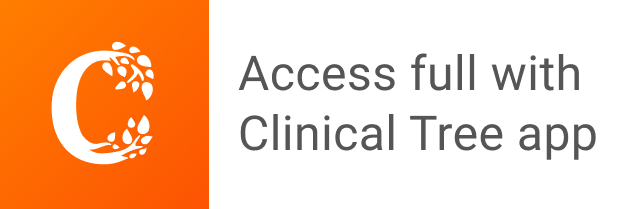