Abstract
Diagnosing inborn errors of metabolism (IEMs) and particularly those complicated by movement disorders means working in a fascinating and overlapping area between neurology, child neurology, metabolic disease, and genetics. At this crossroad between different medical specialties, it is not uncommon that patients enter a long diagnostic journey. Patients can present to a movement disorder specialist, a metabolic specialist, or a clinical geneticist. It is interesting to note that the approach to a diagnosis can be quite different in any of these medical specialties. Neurologists and clinical geneticists often find it difficult to start their diagnostic process in a patient with a suspected IEM with biochemical or enzymatic testing as they are more familiar with molecular testing. On the other hand, specialists for IEMs will often consider biochemical testing their usual starting point to diagnose an IEM.
Introduction
Diagnosing inborn errors of metabolism (IEMs) and particularly those complicated by movement disorders means working in a fascinating and overlapping area between neurology, child neurology, metabolic disease, and genetics. At this crossroad between different medical specialties, it is not uncommon that patients enter a long diagnostic journey. Patients can present to a movement disorder specialist, a metabolic specialist, or a clinical geneticist. It is interesting to note that the approach to a diagnosis can be quite different in any of these medical specialties. Neurologists and clinical geneticists often find it difficult to start their diagnostic process in a patient with a suspected IEM with biochemical or enzymatic testing as they are more familiar with molecular testing. On the other hand, specialists for IEMs will often consider biochemical testing their usual starting point to diagnose an IEM. Because biochemical analytical techniques evolved earlier than the techniques to analyze DNA, historically IEMs were diagnosed by detecting abnormal metabolites or enzyme function. It is important to realize that IEMs are genetic disorders with the majority being autosomal-recessive disorders. Other patterns of Mendelian inheritance, including autosomal-dominant, X-linked, and maternal inheritance, can all occur in IEMs. Moreover, the latter inheritance pattern is specific for IEMs due to mutations in mitochondrial DNA (mtDNA). So in most patients with a suspected IEM, molecular genetic testing can be considered a first-tier test, identical to DNA testing routines in other genetic diseases. However, what makes metabolic movement disorders somewhat different from other genetic movement disorders is that a number of metabolic movement disorders are treatable disorders, and these treatable disorders should be prioritized in the diagnostic approach.
In recent years, the availability of next-generation sequencing (NGS) has changed the landscape of diagnosing inherited diseases, including metabolic movement disorders. One of the reasons for the success of NGS is that it allows a one-step laboratory routine with which can target almost all genetic diseases, not only IEMs, enabling clinicians to cover a wide range of genetic diseases in a single run. The application of NGS in neurology has not only unraveled many novel disease-associated genes, but also has shown that in many metabolic movement disorders a single gene defect can cause a whole spectrum of symptoms. Clinicians using NGS need to have a set of skills to determine how and when to use data, and how to interpret genetic variants in the clinical context. Clinicians need to be aware of the pitfalls and limitations of molecular testing and what to do when NGS does yield a molecular diagnosis. NGS techniques, variant interpretation, potential pitfalls, and a diagnostic strategy that incorporates NGS are discussed.
Sequencing Techniques
Sanger Sequencing
Until a decade ago, Sanger sequencing was the gold standard for detecting mutations in disease-associated genes. This method revolutionized DNA analysis, in particular after automated sequencers became available, facilitating multiple samples to be analyzed simultaneously and delivering test results within a short period of time. In principle, Sanger sequencing consists of determining the sequence of a single gene including intron–exon boundaries and introns, depending on the size of the gene. Rather long reads of DNA can be analyzed, circumventing problems of analyzing genomic regions with great homology, such as pseudogenes. However, when multiple different genes need to be analyzed, multiple single gene tests need to be performed, making this technique time-consuming and hence costly.
Sanger sequencing has not disappeared from diagnostic laboratories as this technique is still the gold standard for disorders of tandem repeats or polyglutamine repeats such as the autosomal-dominant spinocerebellar ataxias, Huntington disease, and fragile X. When just a single gene needs to be analyzed, Sanger sequencing can still be the method of choice. Sanger sequencing is also employed for verification of NGS results.
Next-Generation Sequencing
Next-generation sequencing (NGS) is sometimes referred to as massive parallel sequencing, because this is what the technique does. Small fragments of DNA, usually about 150 base pairs, are multiplied many times and these reads of DNA are aligned by software programs to a standard or reference genomic sequence. For details about NGS, the reader is referred to some excellent recent reviews on this topic [1]. Clinicians need to have an understanding of the NGS techniques offered, including limitations and potential diagnostic pitfalls.
NGS Methods
In diagnostic laboratories, different NGS techniques are used and can include sequencing the patient’s entire genome (whole-genome sequencing, WGS) or the exons of every protein-coding gene (whole-exome sequencing, WES) or targeting specific disease-causing genes (targeted resequencing, TRS or “gene panels”).
Whole-Genome Sequencing
WGS can be used to determine the complete nucleotide sequence of an entire DNA sample, including all the coding and non-coding sequences. The major advantage of this approach is that the complete genomic information will be available and the actual sequencing is relatively fast, since only a short time is required for sample preparation. In contrast to WES discussed below, information from protein-coding sequences does not need to be captured in additional procedures. This is why WGS has been successfully applied for the rapid diagnostic sequencing of critically ill newborns in intensive care units [2], among which of course IEMs are potential diagnoses. WGS is a fast technique, but a major disadvantage of sequencing the whole genome is that it yields a large amount of data that require extensive filtering to provide meaningful information with regard to (known) disease-causing mutations. Therefore, laboratories usually only include and analyze data from the protein-coding sequences, similar to what is done in WES. In addition, WGS as a diagnostic technique is still expensive and there may be problems regarding incidental findings, that is, the identification of potential or definite pathogenic mutations unrelated to the disease for which the genetic test was originally requested. Incidental findings can be encountered in both WGS and WES and raise ethical issues that are discussed later.
Whole-Exome Sequencing
In contrast to WGS, which basically analyzes every single nucleotide of the genome, WES focuses only on the protein coding regions (exons) of the genome. This allows the simultaneous analysis of all the coding regions of known IEM disease genes as well as of novel, potentially disease-causing, genes. To perform WES, first all the coding exons in a DNA sample need to be enriched before the sequence can be analyzed. A major advantage of WES is that all the potential disease-causing genes are included in the analysis: both all the known disease genes associated with movement disorders as well as genes not yet related to a disease. In particular, WES can reveal new disease genes or mutations in genes that have not yet been associated with a certain clinical phenotype. The advantage of WES over WGS is that considerably higher read depths can be achieved at lower cost; furthermore, WES data sets are easier to handle. However, some major concerns for WES include its incomplete representation, in most cases because of low coverage of base-pair reads in certain exons, most often related to insufficient capturing of GC-rich sequences. In the past, incomplete representation and low coverage have led to clinically relevant mutations being missed when WES was used in clinical diagnostics, and therefore these still represent serious quality issues that need to be addressed.
The advantage of being able to test large numbers of genes is sometimes challenged by an incomplete sequence depth. Although capturing techniques have improved, the issues of coverage and sequence depth must still be carefully considered when WES is offered in diagnostic testing. In practice, this means that when a specific disease is suspected and WES did not result in a molecular diagnosis, looking back at the coverage of genes of interest may be advisable.
When a large number of potential disease-associated genes are involved, as is the case in IEMs with more than 800 potential genes, a considerable number of sequence variants needs to be interpreted by laboratory staff. For this reason, most laboratories will apply subpanels of groups of disorders or parent–child trio analysis to reduce the number of sequence variants for interpretation. Trio analysis also facilitates the identification of de novo mutations, which are quite common in neurological disorders, but have not yet been systematically investigated in IEMs.
Despite some of the limitations of WES, it is used in most diagnostic laboratories since it can be applied for the majority of monogenic disorders. The advantage is that all exons are captured and laboratory staff only need adjust filter strategies for different indications. An additional advantage is that new disease-associated genes can be added relatively simply to the gene lists used to filter the data and that new disease-associated genes can be discovered in WES data sets. As with WGS, incidental findings are an issue, as discussed below.
Targeted Resequencing
This third and widely used NGS strategy is to enrich only the coding regions of genes of interest for a specific disease or diagnostic category, for instance all genes for movement disorders associated with dystonia. For TRS, a disease and gene-specific capturing kit needs to be designed and produced. There are some major advantages to restricting the mutation analysis to a limited set of genes: targeted enrichment provides a superior quality of representation and a much higher read depth than that which is usually obtained with WGS or WES. Because the focus is on specific genes, known intronic mutations in these genes can be included in the capture and panel design. For known repeat sequences or structural rearrangements, specific bioinformatics tools can be applied, overcoming some of the major limitations of WES. In addition, as the focus is on known disease genes associated with a certain clinical phenotype, the laboratory staff face fewer challenges in analyzing the data sets and interpreting variants. This leads to shorter turn-around times for test results. Moreover, TRS minimizes the problem of incidental findings. Depending on the platform and the enrichment strategy used, several hundred target genes can be analyzed for multiple patients in a single run. One of the drawbacks of the technique is that gene-specific capturing kits need to be ordered, and adding new disease-associated genes requires a new customized capturing kit requiring new validation of capturing and coverage. TRS can still be a cost-effective alternative to WES, yielding very high-quality sequencing results for movement disorder diagnostics [3].
Variant Interpretation
The basis of all NGS procedures is that extensive filtering algorithms are used to discard sequence variants that are not (or unlikely) associated with disease. This allows selection of a relatively small subset of variants that potentially could explain the clinical phenotype. It is important that clinicians requesting NGS must have some understanding of how these variants are reported and how likely they explain the clinical phenotype. Variants are called pathogenic when the probability that the variant is causing pathogenicity is more than 99%. A variant is called likely pathogenic when the probability is between 90% and 99%, and finally the variant is called a variant of unknown significance (VUS) when the probability of pathogenicity is less than 90% or when no reliable prediction can be made that the variant is indeed benign [4].
Biesecker et al. [5] published an excellent guide for clinicians to apply the data produced by genetic laboratories. They underscore the fact that there is a common misconception that a genetic finding is a deterministic, infallible predictive tool. Clinicians should realize that this is frequently not the case. Pathogenic variants are easy to interpret, but in many cases variants are found that are not predictive of the phenotype, yet can still be useful in making a diagnosis or altering treatment management. The appropriate integration of genetic testing results in comprehensive diagnostic assessments, and neither overestimating nor underestimating the predictive power of genetic analyses is needed to arrive at an accurate diagnosis. So despite the fact that NGS revolutionized our diagnostic procedures, a diagnosis still requires integration and assessment of all available information.
In order to arrive at the correct diagnosis, a knowledge of the clinical phenotypes related to the genes tested is crucial. Therefore, we prefer a multidisciplinary setting where clinicians and laboratory staff discuss the NGS results and interpret variants in relation to the patient’s phenotype. It is important that clinical specialists are involved who have expertise in the disorders tested. A multidisciplinary setting to discuss laboratory results within a team of physicians and laboratory staff is quite common in metabolic laboratories, but this setting is infrequent in laboratories offering NGS diagnostics [6].
Other Molecular Techniques Used in Clinic
Array-CGH and SNP Analysis
Other molecular techniques can contribute to a molecular diagnosis, and this is particularly true for techniques that detect larger structural rearrangements in DNA, such as deletions of genes or even of multiple genes. These techniques, such as array comparative genome hybridization (array-CGH) and single nucleotide polymorphism (SNP) analysis, are quite commonly used in children with developmental delay and intellectual disability. However, although not very often reported in relation to IEMs, complex phenotypes with deletions of (multiple) genes are not uncommon in patients with movement disorders and this is particularly true when developmental or behavioral problems are also part of the phenotype. Dale [7] reported quite a significant diagnostic yield of microdeletions in a group of 7/25 (28%) children with a suspected genetic cause of their movement disorder. Multiple disease-associated genes involved in microdeletions sometimes lead to a contiguous gene syndrome. Well-known examples of these are deletions involving SGCE, causing a syndrome of myoclonus–dystonia, deafness, and split hand malformation [8]. Other examples of such complex phenotypes with involvement of a known movement disorder gene are deletions with congenital mirror movements and deletions involving TOR1A [9, 10].
Interestingly, deletions in the region of a known movement disorder gene can cause the movement disorder phenotype, even when the deletion does not include the gene itself. Adjacent deletions can disrupt gene function, and this has been repeatedly reported for instance in benign hereditary chorea associated with deletions nearby NKX2-1 [11]. Thus, when there is a clear suspicion for a specific gene defect based on the clinical phenotype, it is worthwhile pursuing an array-CGH in search of structural genomic abnormalities.
In many laboratories, SNP analysis will be the technique used to diagnose structural genomic rearrangements. In contrast to array-CGH, SNP analysis is often combined with WES, for instance as a quality-control test. In addition to testing for structural abnormalities, SNP analysis can also be used to test for consanguinity and to identify homozygous regions in search for recessive disorders. Yet another application is to detect uniparental disomy (UPD), which is relevant for imprinted genes such as SGCE.
RNA Sequencing
Sequencing of transcripts or RNA sequencing has been used in DNA diagnostics for many years as a test to find out whether changes/variants in genomic DNA lead to detectable changes in RNA transcripts and hence protein function. When there is no functional test available, i.e. an enzyme assay or transporter assay, RNA sequencing can be used to test the functional relevance of a given DNA change. Massive parallel RNA sequencing allows large-scale transcriptome analysis. Although this technique is not yet available in many diagnostic laboratories, RNA sequencing can be of additional value in a diagnostic setting [12]. Particularly in patients with a suspected mitochondrial disease, a 10% increase in diagnostic yield can be established by performing transcriptome analysis demonstrating intronic loss of functions variants. The diagnostic yield of RNA sequencing may be even higher when the affected tissue is tested. Cummings et al. [13] showed that RNA sequencing on muscle biopsy material significantly increased diagnostic yield as it demonstrated specific splice-altering variants in both exonic and intronic regions that were not detected by preceding WES analyses. It is very likely that RNA sequencing will become available on a large scale in the near future and this will help establish risk scores for more complex genetic disorders.
Diagnostic Yield of NGS Techniques
Depending on the group of disorders and selection of patients, the diagnostic yield of WES or other NGS strategies varies between 15% and 50%, but can be higher in selected patient populations. Bergant et al. [14] investigated, in a large cohort of patients (n = 1059), whether or not additional and extensive analyses on exome data sets could contribute to a higher diagnostic yield. The authors reported that a 4% increase in the number of diagnoses could be established. This was mainly achieved by the addition of a copy number variant analysis and better splice site prediction.
WES only covers about 2% of our DNA. Sequence variants in non-coding (introns) and regulatory domains of the genes of interest are not analyzed and hence not reported. Cordeiro et al. [15] showed that in a pediatric movement disorder clinic the diagnostic yield of NGS techniques could be as high as 50%, with many metabolic movement disorders among these diagnoses. For comparison, the diagnostic yield of cerebrospinal fluid (CSF) diagnostic procedures in about the same population of patients was considerably lower, at about 13% [16]. Reid et al. [17] showed that in 13% of their highly selected cases of undiagnosed neurometabolic disorders more than one gene defect was found to be present, a proportion of patients not to be ignored, and a finding that can only be established by comprehensive genetic testing.
Finally, several groups have shown that NGS as a first-line diagnostic approach can be cost effective. Of note, in a study by van Egmond et al. [18] on the cost-effectiveness of NGS, a patient with glutaric aciduria type 1 and a patient with Niemann–Pick disease type C were diagnosed at ages 44 and 62 respectively, ages at which an IEM as the cause of movement disorders is rarely considered. Adults with metabolic movement disorders are more likely to be detected by NGS.
Pitfalls in the Molecular Diagnosis of IEMs
Despite the fact that NGS offers a powerful technique, there are several scenarios that warrant consideration.
Mutations in Promoter and Intronic Regions
As discussed above, WES will miss mutations in promoter regions and intronic regions. Although these are infrequent causes of IEMs, they certainly exist. In some disorders, for instance in dopa-responsive dystonia and tyrosine hydroxylase deficiency, mutations in the promoter region are well described [19, 20]. This means that in a patient with a clinical suspicion but negative WES, CSF analysis can be very important to arrive at the correct diagnosis. In cases of metabolic movement disorders when one suspects a specific IEM, it can be worthwhile to contact a research group working on this disorder.
Not only mutations in promotor regions but intronic mutations are also well-known, albeit less frequent, causes of IEM and are seen in patients with common IEMs such as phenylketonuria and Wilson disease [21]. We know these intronic mutations exist, again because there is a very good and easy-to-obtain biomarker in blood that allows a diagnosis even if the first-pass genetic test is negative. Therefore, a clinical suspicion for a specific disorder or group of disorders should not be discarded based on negative NGS results. Other ways of confirming the suspected clinical diagnosis should be considered, such as looking for a biomarker or an enzymatic assay, considering a separate analysis for promotor and intronic mutations, or testing for larger intragenic abnormalities as discussed below. In many cases, contacting laboratories that can perform these kinds of additional tests, often in a research setting, can be a way to obtain a molecular diagnosis.
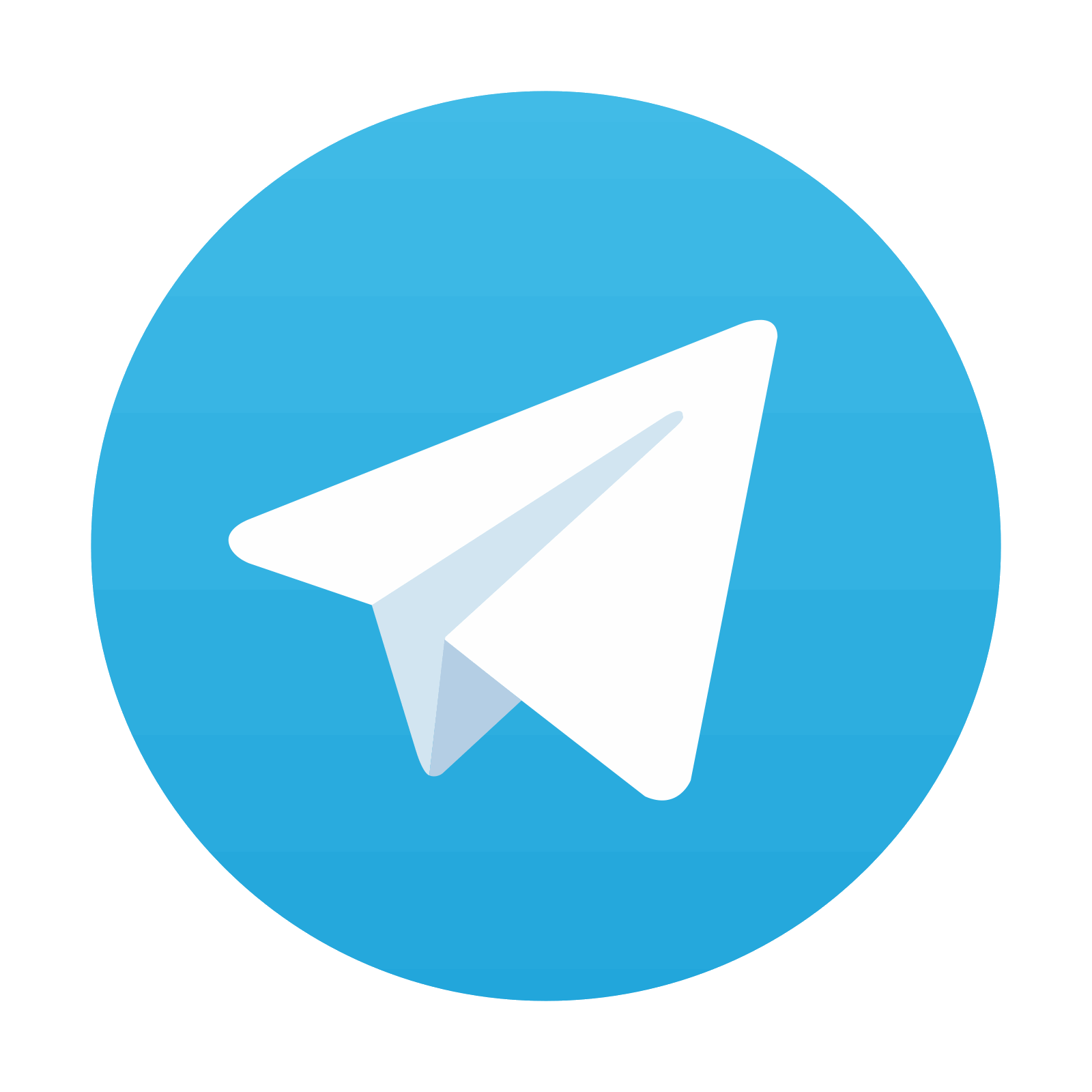
Stay updated, free articles. Join our Telegram channel
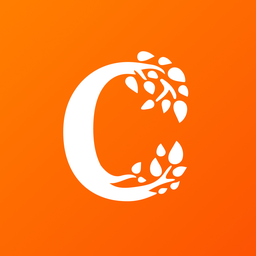
Full access? Get Clinical Tree
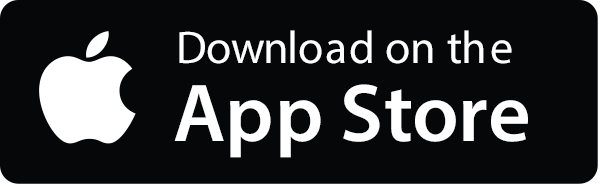
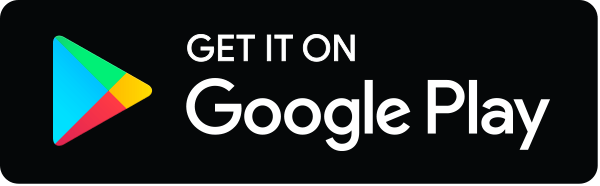
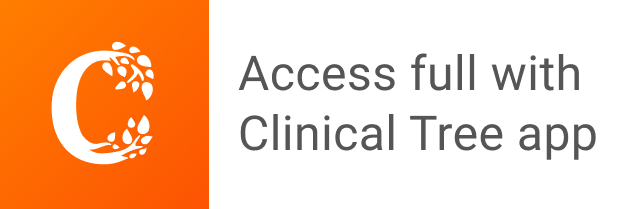