Chapter 7 Electric source imaging in MRI-negative refractory focal epilepsy
MRI-Negative Epilepsy, ed. Elson L. So and Philippe Ryvlin. Published by Cambridge University Press. © Cambridge University Press 2015.
Introduction
Presurgical evaluation of nonlesional epilepsies remains a major challenge. During the last 20–25 years, a number of brain imaging techniques have been proposed and successfully developed, validated first in lesional cases and then progressively applied to patients with nonlesional epilepsy. Nevertheless, in a review of 2010, looking at reports on outcome in lesional and nonlesional epilepsy between 1995 and 2007, only modest numbers of seizure-free patients were retrieved: between 26–46% of all nonlesional patients benefited from surgery, with somewhat better results in temporal lobe epilepsy and in children (1). Integrating other imaging modalities appears therefore vital to increase this success rate. A good example is the study of PET-positive/MRI-negative patients with temporal lobe epilepsy, providing evidence that the lack of a MRI lesion is not necessarily associated with a worse surgical outcome (2).
While there has been major progress in MR-based and nuclear imaging, the use of EEG as a possible imaging technique remains rarely considered, although it is the cornerstone technique in the diagnosis of epilepsy. In fact, even today, most authorities in the field won’t contest that EEG is much valued, not only for the diagnosis of epilepsy, but also for regionalizing the epileptogenic focus. Recent advances in EEG recording and analysis techniques converted the EEG to a valuable imaging tool (3), but these methods have not yet found their place in clinical routine. Plummer et al. (4) reviewed EEG source imaging (ESI) studies up to 2007. They underline the value of ESI and the surprisingly still modest utilization in the presurgical work-up of patients with focal epilepsy. Given that most epileptic patients show epileptogenic discharges on scalp EEG and that EEG source imaging can reliably identify the irritative zone even from standard clinical EEG recordings, its use in clinical routine would be justified. However, similar to MR-machines, “higher power is related to higher yield,” i.e., larger electrode arrays, and full use of EEG-localization algorithms are more likely to be more informative than small arrays and simple approximations.
With the present chapter, we like to expose advantages but also some limits and considerations of electric source imaging (ESI) in epileptology.
Basics of electric source imaging
Electrical source imaging (ESI) is based on the recording of multichannel EEG on the scalp. It relies on mathematical algorithms that estimate the brain sources that give rise to a certain scalp electric field. Since there are many possible solutions for the same electric scalp field, known as the “inverse problem,” a priori assumptions are required to determine a meaningful underlying source or maybe several sources that explain the scalp field. This is true for the EEG as well as its homologous technique, the magnetoencephalography (MEG). The more appropriate these assumptions are, the more likely the inverse solutions are correct and precise.
Assumptions concern the head model as well as the model of the sources that are supposed to generate the scalp fields. The latter gave rise to many studies; their review would go beyond the scope of this chapter. The interested reader is referred to detailed reviews elsewhere (5–8). Other relevant technical aspects of ESI are briefly summarized below.
Head models
The head volume conductor model refers to the shape of the tissue that is supposed to generate the scalp fields, the orientation of the sources in this tissue, and the conductivity properties of the different layers between the active neurons and the scalp electrodes. The most simple head volume conductor model consists of a homogeneous single sphere. By including several concentric homogeneous spherical shells, the different conductivity properties of the tissues (brain, CSF, skull, and scalp) can be “imitated” and included in the calculations. Spherical head models have been used in many source localization studies, particularly in those using equivalent dipole localization methods. They are still very often applied in MEG source imaging studies, including applications to epilepsy (e.g., (9)). Because of their simplicity, spherical head models are computationally efficient. However, the real human head is not a sphere and spherical head models can lead to serious mislocalizations when the solutions are coregistered with the real MRI of the patient (10). Realistic head models that take the real geometry of the individual brain into account lead to improved source reconstructions, particularly in basal brain areas such as the occipital cortex and the mediobasal temporal lobe (11). Realistic head models are more complex and computation-intense, but they allow the direct incorporation of the anatomical structure of the individual patient’s brain from the magnetic resonance images, an aspect that is particularly important in patients with large brain lesions (12). This is particularly important in the context of epilepsy surgery. Realistic head models are based on boundary or finite element methods with the latter being able to incorporate also inhomogeneous conductivities of the head such as the anisotropic conductivity distribution of the white matter (13). Realistic head models also allow the delineation of the sulci and gyri in the brain, and thus allow the restriction of the local orientations of the dipoles (14), thereby reducing the number of possible solutions.
Source models
The source model refers to the assumptions about the current sources in the brain that are responsible for the scalp potentials. It is generally assumed that the primary sources of the EEG are the postsynaptic currents of a large number of pyramidal cells that are simultaneously active. Since the EEG electrodes on the scalp are far away (far field) from these neurons, electrical activity can be modeled as an equivalent current dipole. If only one or a very few current dipoles are assumed, their most probable location for a given momentary scalp field can be determined by iterative procedures. Such simplified equivalent dipole models have been most commonly used in the initial EEG source imaging studies and is still the method of choice in MEG source imaging in epilepsy (15). However, equivalent dipole models are only valid if the neuronal sources are confined to a few focal regions and if the number of these regions are known a priori. If multiple sources distributed over the entire brain volume are simultaneously active, the equivalent current dipole model may lead to erroneous results.
Distributed current source models have been developed that can account for the whole-brain bioelectric activity without a priori assumptions on the number of sources. Distributed current source models parcel the whole brain in small regions and position a current dipole in each of these regions (solution point). These solution points can be restricted to the gray matter of the brain, if realistic head models are used. Since the number of solution points is generally much larger than the number of measurement points, additional constraints have to be imposed in order to obtain unique and well-posed linear inverse solutions. The first proposal to solve this inverse problem was termed the minimum norm least-squares (MNLS) inverse that minimizes the least-square error of the estimated inverse solution (16). Variations of the MNLS were developed by including the dependencies of a local point on the activity of its neighbors. In other words, neighboring neurons behave most likely more similar than neurons in remote regions. Such additional a priori information is included in algorithms known as low-resolution brain electromagnetic tomography (LORETA) (17), variable resolution electromagnetic tomography (VARETA) (18), local autoregressive average (LAURA) (19), and others. Also, methods to assess the statistical significance of the inverse solutions have been developed such as dynamic statistical parametric mapping (dSPM) (20) and standardized LORETA (sLORETA) (21). Most of the recent applications of EEG source imaging in epilepsy use these linear distributed inverse solutions.
Number and position of electrodes
It is evident that the precision of EEG source imaging not only depends on the proper head and source model, but also on the proper sampling of the electric field over the whole scalp, including basal brain areas to better estimate the activity of the inferior temporal or orbital frontal cortex (22) (Figure 7.1). Electrodes also have to be spaced properly to avoid spatial undersampling (23). The spatial frequency, intimately associated with the precision of ESI, is limited by the blurring induced by the low conductivity of the skull (24). Early simulation studies estimated the maximal spatial frequency – or the distance between electrodes – to 1–2 cm (25), requiring more than 100 electrodes to cover the whole head. Downsampling approaches on data sampled with high density confirmed this number, showing severe localization errors of known epileptic foci when the electric field was sampled with 64 electrodes or less (26). Moreover, simulation studies by Ryynänen et al. (27) showed that by using more realistic conductivity values for the skull, the number of electrodes should be rather above 200. While the application of these high number of sensors is obviously more challenging for EEG than MEG, recent advances in EEG technology make it possible to record from high-density EEG with reasonable efforts (28, 29).
Figure 7.1 Illustration of the electrode positions of a high-density EEG (in this case a Geodesic Net from EGI Inc.) in comparison to the position of electrodes in the standard 10–20 system. Left: electrodes’ position revealed by measuring the subject’s MRI with the net in the scanner. Middle and right: electrodes’ position with respect to the underlying segmented brain MRI. The lower rows show zoomed-in regions. Note the large areas of the brain on temporal and medial brain areas not covered by the 10–20 system
Electric source imaging in focal epilepsy
In recent years several studies demonstrated that ESI could be a very useful tool in the clinical work-up of patients with focal epilepsy, not only to localize the epileptogenic zone, but also to visualize the propagation of ictal and interictal discharges within the epileptic network (for reviews see (4, 6)). Even when applied to standard EEG recordings performed in the clinical settings, i.e., with only 25–35 electrodes, it can provide important hints with respect to the focus localization at a lobar level and guide the positioning on intracranial electrodes (22), if an invasive procedure is felt necessary. The advantage of ESI coupled with clinical long-term EEG is that it allows recording and analysing interictal activity in patients with rare spikes or spikes only appearing during sleep.
However, for the reasons explained above, ESI is most powerful when the EEG is recorded from a high number of electrodes. In this case, the clinical yield of ESI exceeds or equals that of conventional presurgical imaging methods PET, SPECT, and MRI as demonstrated in a recent prospective study of 152 operated patients (30). All patients had a sufficiently long (> 1 year) postsurgical follow-up to reliably assess the postoperative seizure outcome, allowing the evaluation of the sensitivity and specificity of ESI. If the EEG was recorded with a large number of electrodes (128–256 channels) and if the individual magnetic resonance image was used as head model, a sensitivity of 84% and a specificity of 87% of ESI were obtained. These values were superior to those of structural MRI (73% sensitivity, 50% specificity), PET (65% sensitivity, 37% specificity), and ictal–interictal SPECT (54% sensitivity, 62% specificity). The sensitivity and specificity of ESI decreased to 73% and 75%, respectively, with a low number of electrodes (21–29 channels). Lowest values were found when low-resolution EEG was combined with a template head model (59 and 62%, respectively) (Figure 7.2).
Figure 7.2 Sensitivity and specificity of localization of the epileptogenic focus with different imaging methods. Data from 52 out of the 152 operated patients who underwent all investigations. Sensitivity is defined as percentage of patients with the maximum abnormality being located within the operated area when the patient became seizure-free after surgery. Specificity is defined as percentage of patients with maximum abnormality lying outside the operated area when the patient did not become seizure-free. HR ESI = high-resolution ESI (128–256 channels), LR ESI = ESI with clinical EEG (< 29 channels), i-MRI = individual head model for ESI, t-MRI = template head model for ESI, MRI = structural magnetic resonance imaging, PET = positron emission tomography, SPECT = single photon emission computed tomography.
Electric source imaging in nonlesional focal epilepsy: impact on surgery
It is evident that ESI is an excellent imaging tool for patients with nonlesional epilepsy, given that MRI, the other cornerstone exam, is negative. In a recent study of our group, we looked at yield of ESI in ten patients with normal MRI (31). Ictal EEG data and seizure semiology pointed to an epileptogenic zone located in the temporal lobe in five patients, in the frontal lobe in two, and in the temporo-occipital, parieto-occipital and frontotemporal regions in the remaining three. Presurgical evaluation included long-term video-EEG, PET, interictal, and ictal SPECT in all patients, and invasive recordings in eight of them.
In five of the ten patients ESI was performed on their standard clinical EEG consisting of 29–31 electrodes. In the other five patients high-density EEG with 128 or 256 channels was recorded, and ESI was based on the spikes detected in these recordings. A simplified individual head model based on the patient’s MRI was used in all cases. In order to define the accuracy of the ESI, the results were coregistered with the postoperative MRI and the ESI was considered correct when the maximum fell within the resected area.
The analysis revealed a localization of the ESI maximum within the resected zone in eight of the ten patients (Figure 7.3). All of these patients benefited from the surgery: six were seizure-free, two had an Engel class II outcome. In contrast PET and SPECT provided localizing results in only half of the patients. One of the two patients with presumably incorrect ESI localization suffered from persistent seizures after surgery. The ESI maximum in this case was localized adjacent to the resected zone (see also below, case 3).
Figure 7.3 Epileptic focus localization with ESI in patients with nonlesional focal epilepsy. The yellow-green area indicates the ESI maximum superimposed on the post-operative MRI when available or on the preoperative MRI with the approximated operated zone marked by the red dashed line. The source maximum laid within the resected area in eight patients and outside in two [1 and 7]. One of them [1] was not seizure-free after operation.
The second patient with incorrect ESI localization was seizure-free after surgery. In this case, the scalp EEG only recorded spikes from the inferior parietal lobe, while the intracranial recordings found interictal spikes in two regions, the inferior parietal and interhemispheric parietal cortex. Thus, ESI correctly localized the inferior parietal spikes. These spikes persisted in the postoperative scalp EEG, but this focus fortunately did not provoke seizures (follow-up: 3 years).
It is interesting to note that the histopathological examination of the resected tissue showed abnormalities in all cases: diffuse gliosis in five patients, cortical dysplasia in three, microheterotopies in one, and a combination of these abnormalities in the remaining patient. This indicates that patients with MRI-negative results are not entirely nonlesional. It might be that in some of these patients a re-evaluation of the MRI, guided by the ESI result, would have identified small lesions. Such findings have been reported in an interesting recent MEG study of 29 nonlesional epileptic patients (32). They showed that in seven of these 29 patients MEG-guided re-evaluation led to the identification of clear lesions that were previously unidentified (see also case 4).
Localization of ESI, or dipole orientation of the source, could have also an impact on the precise focus localization or even on the postoperative prognosis. For example, nonlesional temporal lobe epilepsy might be related to different generators compared to TLE associated to hippocampal sclerosis (HS). It was hypothesized that patients with HS have spikes with oblique equivalent dipoles while patients with discrete cortical lesions have spikes with radial dipoles (33). This has been questioned by other reports, which found no differences in a group of patients with mesial TLE (34, 35). In a more recent study (36), the dipoles of 12 nonlesional cases and 22 patients with hippocampal sclerosis were compared using 29 scalp electrode recordings and realistic head models. No difference was found, with around 25% of each patient group showing localization in the mesial temporal structures. Furthermore, no difference between patients with good and poor outcome was found (HS+ and HS- patients were distributed equally in both groups). Thus, measuring the orientation of the dipole does not seem to provide additional relevant information, at least if based on an EEG with < 60 electrodes.
Is ESI reliably localizing deep structures?
There is still ongoing discussion if noninvasive electromagnetic tools are able to depict deep foci, e.g., in mesial temporal or extratemporal structures. Temporal deep structures probably behave differently than extratemporal deep sources, given that the electric field is generated predominantly by tightly packed parallel oriented pyramidal cells in the hippocampal layers. This creates a relatively clear dipole or electrical field, which should be picked up by scalp electrodes 3–4 cm away. Some authors claim that mesial temporal structures are only “seen” if they recruit simultaneously lateral temporal neocortex and that neither EEG nor MEG is able to detect spikes confined to the mesial temporal structures (37, 38), whereas others showed data that suggest the possibility to localize deep temporal foci from scalp recordings, particularly if the activity (spikes or evoked responses) is averaged (39–42). The demonstration of disappearance of spikes on the scalp EEG after selective mesial resections indicates that mesial spikes can be localized by ESI (43). However, the controversy might never be settled given that it is difficult to record simultaneously with high spatial sampling from inside the hippocampus, the lateroanterior temporal cortex and from the scalp. Combined recordings of EEG-fMRI or simultaneous high-density EEG/MEG could provide further insights on this issue (44–46).
Do we need spikes to localize the epileptogenic source?
Spikes, sharp waves with or without consecutive slow waves, are the hallmark of the diagnosis of epilepsy. Fortunately, in most patients, they are present and allow determining if a focal disorder is at stake and which lobe or region is most likely affected. However, in some cases, no epileptiform discharges are detected. Even in intracranial recordings, there might be no clearly identifiable ictal EEG onset, especially if originating in the interhemispheric region. Thus not even invasive monitoring can be considered as the gold standard (47). Personal observations also showed that intracranial electrodes might not pick up epileptiform activity if displaced 1–2 cm from the epileptogenic zone, underlying again the need for powerful preimplantation localization procedures.
One way to overcome “spike-free” recordings is the acquisition of combined high-resolution EEG and fMRI. In a recent study, scalp voltage maps were computed from previous epileptogenic discharges, then retrieved in the 64- or 128-channel EEG obtained during the fMRI, which then allowed the identification of BOLD changes in relation to the epileptogenic maps. This led to the correct localization in 14/18 patients (78%) with EEG-negative fMRIs (46). However, this requires the recording of spikes at some point. If spikes or other epileptogenic discharges were never obtained, but an epileptic focus is strongly suspected, “template” maps of the presumed focus and the calculation of their presence, as indicated above, could be a solution. However, the reliability of this approach remains to be demonstrated.
Illustrative case studies
Mixed studies, i.e., studies including patients with temporal and extratemporal epilepsy, report equally good localization performance of ESI in both groups. Here we present several cases, which show the advantages but also the limits of ESI in the individual patient.
Patient 1 is a left-handed 18-year-old male patient, otherwise healthy and cognitively unimpaired, who suffered from drug-resistant seizures since the age of 8. Clinically, the seizures were characterized by loss of contact, grunting, and automatisms of the mouth and hands lasting 30 to 60 seconds, followed by amnesia for the event. There was possible postictal aphasia. The MRI was normal but PET was suggestive of a left anterior temporal hypometabolism. In the 32-channel video-EEG monitoring, interictal discharges were most often found over the anterior left temporal lobe (50%), with less frequent posterior temporal (25%) and right-sided temporal epileptic discharges (25%). Ictal onset appeared to be left hemispheric, probably temporal, but unambiguous localization or lateralization could not be obtained in this patient, who also presented evidence of right-sided language lateralization.
An ESI was performed using the predominant left anterior temporal spikes, which localized the source precisely to the anterior aspect of the mesial temporal lobe structures.
Invasive video-EEG monitoring was performed with subdural grid and strip electrodes on the left hemisphere, targeting mainly the left temporal lobe, with depth electrodes inserted in both hippocampi. The seizure onset zone coincided with the contacts superposing with the ESI zone (Figure 7.4). This case illustrates the capacity of ESI to localize mesial temporal foci. Furthermore, an ESI of interictal spikes provides valuable information of the ictal onset zone, since it often coincides with the contacts of intracranial ictal onset (> 60%, personal unpublished data).
Figure 7.4 Case 1 (left temporal nonlesional epilepsy). ESI of the most frequent interictal epileptogenic discharges found the source maximum in the left hippocampus (red cross). The purple contacts of the left hippocampal depth electrode were involved in generating the interictal spikes (irritative zone) and seizure onset and coincided with the ESI source. This case shows the capability of ESI to identify deep temporal sources.
Resective surgery was carried out 2 years ago and the patient is since seizure-free.
Patient 2: The fact that ESI performs equally well in extratemporal nonlesional epilepsy is illustrated by this case (48). This is a 6.5-year-old girl suffering from focal epilepsy since the age of 4.5 years. She presented an undefined, unpleasant feeling, followed by hypersalivation, left arm elevation and right arm extension evolving to a fencing position or tonic abduction of arms with postictal aphasia. Secondary generalization was frequent. The seizures were almost exclusively nocturnal, up to 20 per day. The interictal EEG showed a very active left frontotemporal lobe focus. An ESI was performed on these interictal discharges, indicating a left opercular source, concordant with PET and SPECT findings (Figure 7.5).
Figure 7.5 Case 2, a 6.5-year-old girl with extratemporal nonlesional epilepsy. MRI was normal, but ESI (green), ictal SPECT (blue) and PET (focal hypometabolism, not visible due to superimposition of ESI and ictal SPECT) pointed to the left frontal opercular–insular region. Due to the high convergence of data, and despite the proximity of Broca’s area, the child was successfully operated without intracranial monitoring
The question was arising if intracranial monitoring should be performed. However, we decided to proceed directly to resective surgery for several reasons: (1) there was no other candidate region for the origin of seizures, (2) there was a high likelihood for incomplete electrode coverage of this region, (3) language mapping was not necessary, since we knew already that language was nearby (postictal aphasia), and also not possible because she spoke only Albanese, (4) she would most likely recover from postoperative aphasia given her young age.
The resection was performed using intraoperative monitoring. She suffered from postoperative Broca’s aphasia and right brachiofacial paresis, from which she recovered within 6 months. She is seizure-free since 3 years. Histopathological examination revealed cortical dysplasia.
This case illustrates that good results from ESI are not confined to temporal lobe epilepsy. Noninvasive imaging in extratemporal nonlesional epilepsy could lead directly to surgery, without intracranial monitoring, provided that all image modalities including ESI are concordant.
Patient 3: The seizure disorder of this 9-year-old girl started at the age of 3.5 years. The semiology was stereotypic, i.e., a sensory aura with painful features of the right foot, followed by a Jacksonian march towards the upper limb, and hypermotor seizures with extension of all four limbs. The interictal EEG showed intermittent slowing, occasionally with sharp features, over the left superior parietal region; the ictal EEG had diffuse onset or delayed left centroparietal slowing. The PET and MRI were normal and ictal SPECT showed hyperperfusion in the left basal ganglia. Taking the semiology and all examination findings together, it appeared that the girl suffers from left hemispheric extratemporal lobe epilepsy, either close to the primary sensory foot cortex or, to the posterior opercular-insular region, given the pain component of her aura.
An ESI was obtained based on the suspicious left superior parietal EEG features but without clear epileptogenicity. The results indicated a source in this region, postcentrally and simultaneously a weaker activity in the temporal neocortex (reflecting the posterior opercular region) (Figure 7.6).
Figure 7.6 9-year-old girl who underwent extensive evaluation for her MRI- and PET-negative left hemispheric epilepsy with sensory, somewhat painful foot auras. ESI solutions (green) and resected volume (red) superimposed on the MRI with the positions of the subdural electrodes (blue dots). The yellow star indicates the interictal and (delayed) ictal onset. The ESI identified two sources: a strong superior parietal and a weaker left deep temporal source. The patient continued to present seizure postoperatively, either because of insufficient resection leaving the ESI in place, or because the ictal onset zone was in fact remote in the deep opercular cortex.
Intracranial recording was performed consisting solely of subdural electrodes covering the central and posterior left hemisphere. Additional depth electrodes targeting the deep opercular or insular regions was strongly suggested, but considered too dangerous by the neurosurgical team at that time. Sixteen habitual seizures were recorded with delayed EEG onset after clinical onset in the superior parietal cortex, probably reflecting secondary recruitment of the symptomatogenic zone.
Consequently, resective surgery was not proposed. However, due to insistence of the family, and in the context of a severe epilepsy with up to 30 seizures per day, surgery was carried out as palliative treatment, i.e., a very circumscribed resection of superior postcentral cortex, next to but not including the ESI site. A few weeks after the intervention, the seizure recurred with a similar frequency. The histopathological exam was unrevealing.
This case shows that ESI can map any EEG feature, but only the clinical review of all data will finally determine if a source “makes sense.” The ESI localized correctly the origin of the sharp slow waves, but several aspects in her history made a primary superior parietal focus unlikely. We do not know at this point if the resection was too small, because it left the ESI maximum outside, or if ESI on slow waves, even if it has sharp features, is inadequate for accurate presurgical localization of the epileptogenic zone.
Patient 4: The 10-year-old boy, right-handed, started at the age of 4 with nocturnal nonlateralized hypermotor nocturnal seizures, sometimes preceded by paresthesias in the left hand or face. Noninvasive monitoring showed a very active interictal focus with rhythmic spikes or sharp waves of right central maximum. The ictal EEG did not show focal or lateralized changes, but with a delay of 10 seconds, right frontal discharges. The MRI was normal, ictal SPECT, ESI, and PET identified a right inferior postcentral focus. Given the proximity to primary sensory cortex, we carried out an ESI of somatosensory evoked potentials (SEPs, air puffs of the thumb).
Invasive monitoring confirmed the hypothesis of right postcentral epilepsy. Interestingly, SEPs from the intracranial electrodes and corticography showed excellent concordance with preoperative SEP ESI (Figure 7.7).
Figure 7.7 10-year-old boy with right postcentral epilepsy. Retrospective MR review identified a suspicious cortical area in the deep basal postcentral structures. Upper row: PET (yellow) showed a suspicious area of hypometabolism despite presence of cortex. 1 cm above ESI maximum (red). Lower row: results of corticography allowing the identification of sensory hand cortex (blue rectangle) and hand motor cortex (red rectangle). These findings coincide with ESI of somatosensory evoked potentials obtained preoperatively (green) and with evoked potential recordings from intracranial electrodes (blue dots). The epileptogenic zone is depicted by the light blue dashed circle, close to the vital cortex but not superimposing it.
The child underwent a right inferior postcentral cortical resection, sparing the hand sensory cortex. No more seizures occurred for 2 years since surgery.
This case illustrates again that concordant noninvasive imaging, including ESI, is able to localize precisely extratemporal lobe foci. Moreover, ESI of evoked potentials, obtained with large electrode arrays, identifies relevant cortex, which helps to shorten the cortical stimulation sessions, particularly important in children and patients with limited collaboration.
Electric source imaging in MRI-negative focal epilepsy: impact on identification of relevant pathological networks
While the major application of ESI is in the field of epilepsy surgery, it is also used in patients who are not candidate for surgery, but suffer from focal epilepsy, or presumably focal epilepsy. Epileptic encephalopathy with continuous spikes and waves during slow wave sleep (CSWS) is an age-related epileptic disorder characterized by acquired neuropsychological impairment or even mental retardation, heterogeneous seizure types, and subcontinuous interictal spike-wave activity in the EEG during slow wave sleep. There are different etiologies, which have in common that they include focal pathologies, symptomatic or nonlesional. However, since they are characterized by a nonfocal EEG pattern, lateralized or generalized, network alteration needs to play a role to explain this discordance.
A combined EEG-fMRI and ESI study was done in 12 children with CSWS, all with negative MRI or subcortical abnormalities such as periventricular leukomalacia (49). Interestingly, an ESI of the initial activity points to the perisylvian–insular region in all patients who showed a focal onset of their spike sources (8/12). These were right-sided in two and left-sided in six patients, indicating that there is a focal component despite the rather diffuse and generalized EEG pattern. In comparison, the simultaneously acquired fMRI provided less focal results, due to poorer temporal resolution, but in all patients some of the BOLD changes were also found near or in the perisylvian cortex.
Patients with genetic mutations are usually not considered for epilepsy surgery, except those with tuberous sclerosis who are lesional in essence. An example of successfully operated nonlesional genetic epilepsy is provided by Weckhuysen et al (50). In the context of a larger genetic study of patients with early-onset epileptic encephalopathy, they described a 2-year-old girl with a STXBP1 mutation and focal seizures originating in the right posterior cortex. Intracranial recordings showed multifocal onset but confined to the right temporo-occipital cortex, subsequently leading to occipital lobe disconnection and subpial transections of the temporal lobe. Histopathology showed dysplasia type 1a. The girl enjoyed a 95% seizure reduction. An ESI was not performed in this patient. This case underlines indications of resective surgery beyond the classical MRI-negative epilepsy, i.e., with a known genetic mutation. Larger studies in the field of epilepsy surgery in genetically determined epilepsy syndromes are needed. Furthermore, the potential for ESI to help in localizing the surgical focus and in improving surgical outcome also needs to be studied in such cases.
Conclusion
Electric source imaging (ESI) is an elegant tool to localize the epileptogenic cortex in the individual patient. If combined with realistic head models, i.e., of the patient’s own MRI, and high count channel numbers (> 100), the precision is quite high with a sensitivity and specificity of > 80%. However, it requires the identification of clearly epileptogenic EEG pattern in a given patient, i.e., it does not make knowledgeable EEG readers obsolete! Combined with good-quality PET or ictal SPECT studies, careful patient’s history taking and examination, the rather poor prognosis of MRI-negative epilepsy can be significantly improved, in particular if all results are coconcordant.
As shown with the third case report, it is not sufficient to localize an ambiguous epileptogenic EEG pattern. An ESI can localize any EEG component, so clear guidelines are required to avoid mislocalization. The EEG pattern might not be always spikes or spike-wave complexes, but could include rhythmic slowing or sharp slow waves if it is felt that they reflect directly the patient’s epileptogenic focus. However, it is mandatory to capture enough epileptogenic discharges. In that respect, ESI is probably superior to EEG-fMRI or MEG, which are usually more time restricted. Newer high-resolution EEG machines also allow long-term monitoring for several hours or days, so discharges can be recorded more easily and more frequently. More prospective studies are needed to determine if other aspects of ESI, e.g., propagation pattern, frequency of the presence of epileptogenic focus sources, or ictal ESI studies, combined with noninvasive vital cortex localization, will decrease the need for invasive monitoring, and improve the postsurgical seizure outcome of nonlesional focal epilepsy to a level similar to that of lesional epilepsy.
References



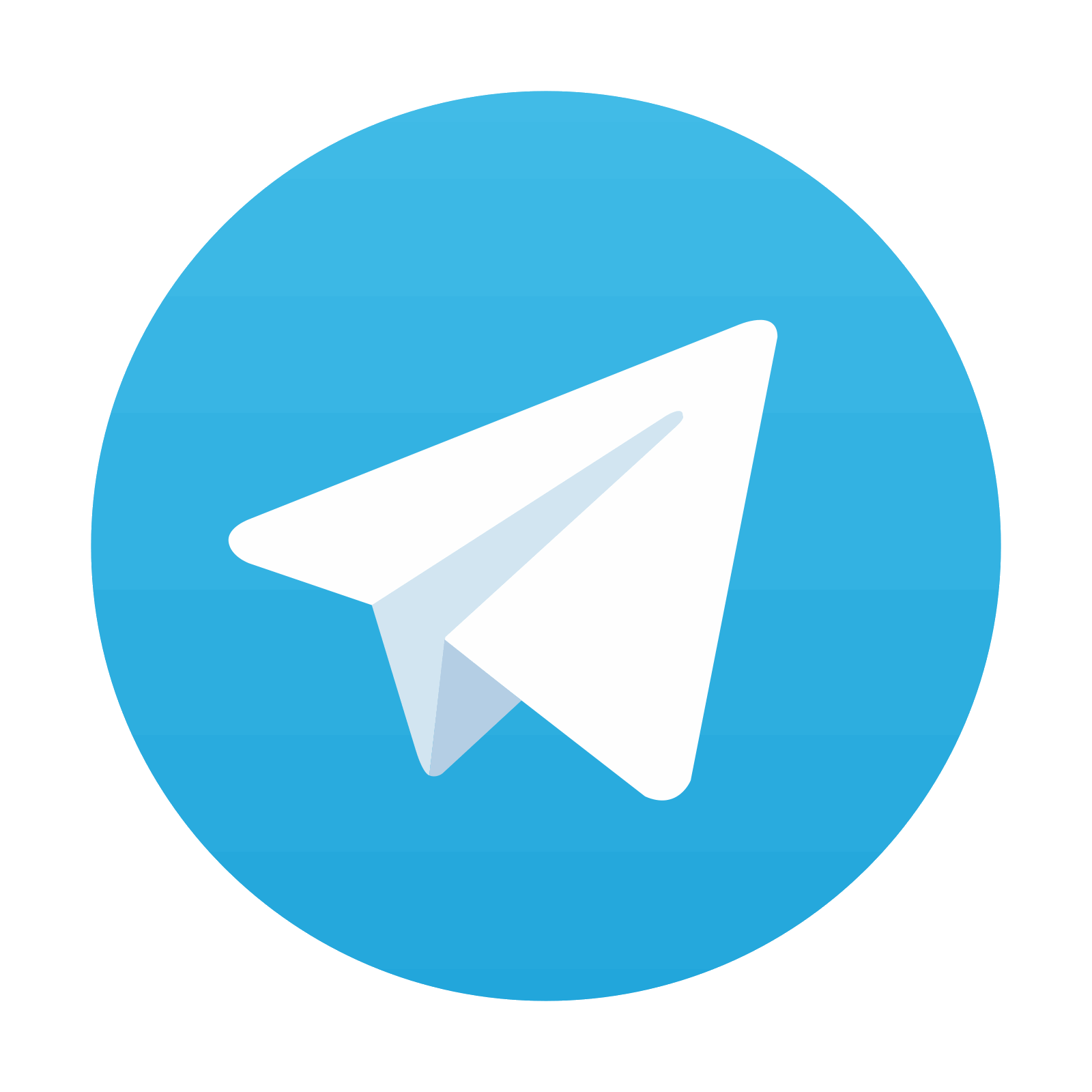
Stay updated, free articles. Join our Telegram channel
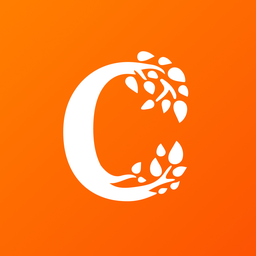
Full access? Get Clinical Tree
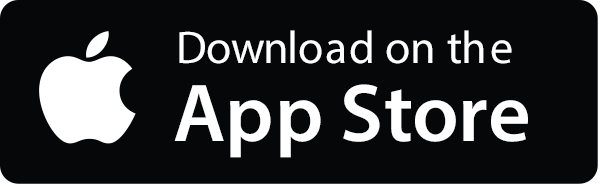
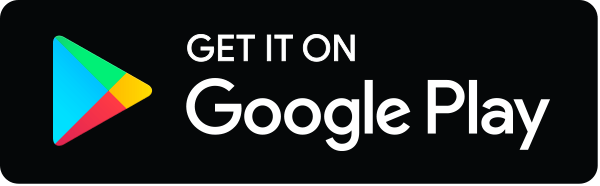
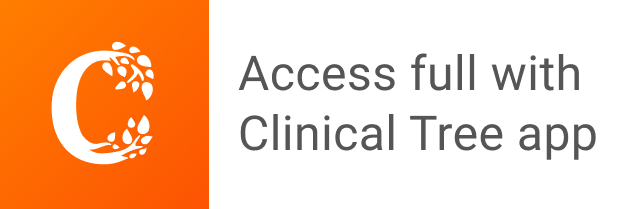