Chapter 8 Functional MRI in MRI-negative refractory focal epilepsy
MRI-Negative Epilepsy, ed. Elson L. So and Philippe Ryvlin. Published by Cambridge University Press. © Cambridge University Press 2015.
Abbreviations
BOLD – blood oxygenation level dependent; EPI – echo planar imaging; EEG – Electroencephalography; fMRI – functional magnetic resonance imaging; HRF – hemodynamic response function; IED – interictal epileptiform discharge; SOZ – seizure onset zone; icEEG – intracranial EEG; MREG – magnetic resonance encephalography.
Mapping of eloquent areas
Presurgical evaluation of eloquent areas is essential in patients with MRI-negative, drug-refractory epilepsy. Especially in children, craniotomy and wake cortical mapping might not be an option to preserve language and memory function, which are at risk in dominant hemisphere surgeries. Therefore, additional tools are needed to reliably depict eloquent brain areas prior to surgery to avoid postoperative deficits.
Wada testing
To avoid such deficits, the intracarotid amobarbital (Wada) test has been used for decades (1). However, this procedure comes with a risk of stroke or other adverse consequences of sedation due to the amobarbital itself. The sensitivity can be reduced, if there is cross-flow of blood to the opposite hemisphere via the circle of Willis, which is why the catheter has to be advanced further into more distal vessels.
fMRI
Functional MRI (fMRI) was introduced two decades ago, in order to noninvasively depict functional areas of the brain (2). Activated areas need more oxygen during task performance, which results in increased blood flow in these areas. This leads to a local signal drop in T2*-weighted images in activated areas, which can be measured as blood oxygenation level dependent (BOLD) signal changes. In order to map such BOLD changes, at least two conditions are necessary in a classic block design to differentiate between activation and resting state. Usually, several periods of activation are performed using echo planar imaging (EPI) in order to enhance sensitivity. More advanced methods include event-related designs (see the EEG-fMRI section in this chapter). The BOLD signal changes are statistically analyzed, color-coded, and overlaid on to the anatomical MR images. Jack and colleagues applied fMRI clinically for the first time: fMRI of the sensorimotor cortex prior to surgery was validated intraoperatively with electrophysiological techniques and has since then become state-of-the-art mapping in many centres prior to surgery of tumors in or adjacent to the motor strip (3).
fMRI reliability
The distance of BOLD signal activation and the edge of planned resection seems to be crucial with respect to possible postoperative neurological deficits. Haberg et al. found that there were fewer postoperative deficits, if this distance was more than 10 mm in brain tumor surgery (4). This was further underlined by Krishnan et al., who demonstrated that a lesion-to-activation distance less than 5 mm resulted in new postoperative neurological deficits; he therefore recommended direct cortical stimulation below a distance of 10 mm from the tumor border (5). One downside to this approach, however, is that the size of the activation cluster depends on thresholding the data; therefore, the determination of a “safe resection” can not be made based only on fMRI. The same holds true for laterality in language so that any laterality index should be independent of thresholds (6–8). Furthermore, a problem in language mapping only using fMRI is that fMRI activation patterns depend on the language tasks. Thus areas not activated by a specific task might be resected, which might result in a postoperative decline. On the other hand, less dominant language areas might not be removed based on fMRI results, which may result in inadequate seizure control (9). Further limitations of fMRI are that cooperation of the patients is needed and that the patients need to be able to perform the required tasks. Also, fMRI does not measure neural activation but merely a vascular response of the capillary bed and draining veins (10), even if a close topographical correlation was found in the macaque (11).
The fMRI and Wada for language and memory mapping
The reliability of fMRI compared to the Wada test has been investigated in a large number of studies showing good concordance (12). However, fMRI depicts areas of the language network which can involve both hemispheres (7). Binder and coworkers presented a method to define language dominance based on a laterality index by counting activated voxels in each hemisphere (13). Lateralization of language seems to be more reliable when using tasks like sentence or word generation (14). Even if fMRI for the purpose of language lateralization is widely accepted for presurgical evaluation of language function, there is still doubt about its reliability (see above). Sabsevitz and colleagues were able to show that preoperative fMRI can predict naming outcome in patients who were to undergo left anterior temporal lobectomy (15). The laterality index based on fMRI tends to be a stronger predictor for postoperative outcome (poor naming) than the Wada testing laterality index. This is in line with several studies that have demonstrated limitations of the Wada test in predicting verbal memory decline after anterior temporal lobectomy (16–18). Using a scene-encoding paradigm that leads to bilateral activation, Binder and colleagues analyzed activation in the hippocampus and adjacent areas and found an asymmetry depending on the side of seizure focus: especially the anterior hippocampus laterality index which showed clear evidence of functional lateralization away from the side of the seizure focus, as expected in chronic temporal lobe epilepsy. However, unlike language lateralization, the hippocampal activation asymmetry was unrelated to postsurgical verbal memory outcome (19). A recent study presented data from language lateralization by fMRI and Wada testing in 229 patients with epilepsy. It again showed a good concordance between fMRI language lateralization and Wada testing. The degree of rightward shift of language dominance on fMRI testing was strongly correlated with Wada/fMRI discordance, suggesting that fMRI may be more sensitive to right hemisphere language processing than Wada testing is (20).
Studying memory in functional MRI is challenging. Using different paradigms, many aspects of memory can be investigated (e.g., encoding and retrieval of patterns, words, faces), which show activation in different areas. Studies investigating the retrieval of items demonstrated medial temporal lobe activation with slight variations (21–23). Left-sided activation was found with verbal stimuli, and bilateral activation was detected with pictorial stimuli (24–26). Studies on memory and language fMRI studies performed to predict postoperative decline in verbal memory following temporal lobectomy indicate that individuals with greater ipsilateral activation compared to contralateral mesial temporal activation have a greater decline in memory following temporal lobectomy (16, 27, 28). Dupont and colleagues demonstrated that fMRI activation during a delayed-recognition task seems to be a better predictor of postoperative verbal memory outcome than the Wada test (29).
fMRI in children
fMRI in children faces further challenges. Some studies suggest that the hemodynamic pattern of fMRI differs in children (30). Tasks have to be adapted to the cognitive abilities, and a passive stimulation paradigm not requiring any particular response may be needed in younger children (31). In a younger age, there is more widespread activation (32) with age-related changes in different regions (33). The children’s attention needs to be focused on the task and monitored during the experiment, especially when cognitive tasks are involved. A rest condition during fMRI is even more problematic in children than in adults, as it is hard to verify in children. Furthermore, the brain scan environment is perceived by many children as frightening, especially because of the required immobilization of the head. Therefore children should become accustomed to the scanner prior to any experiment and the study itself. Yuan et al. showed that introducing visual inputs to the patient reduce head motion (34).
Plasticity of eloquent areas
The developing brain is characterized by a large degree of plasticity. Dehaene-Lambertz et al. demonstrated a left-dominant activation of language networks in children at as early as 3 months of age before any language development seems to begin (35). There is an ongoing discussion about whether language-related areas are shifted to the right hemisphere in early left-sided brain injury or epilepsy (36, 37), or whether there is an intrahemispheric shift (38). The pattern or reorganization might depend on various factors, such as handedness, type of the lesion, age at onset, or duration of the symptoms. Subtle deficits in language performance in children with lesions in the left hemisphere might suggest a left-sided dominance of language (39). Lesions acquired before the age of 5 years might lead to reorganization but it has been shown that language areas often remain in their determined location even in early-onset seizure patients. However, a complete shift of language can also occur when the lesion occurs very early in life (37, 40). Studies have demonstrated that patients with early onset of epilepsy undergoing anterior temporal lobectomy have a lower risk of experiencing a decline in postoperative language (41), which again underlines the idea of a contralateral shift of these regions (42).
EEG-fMRI
Scalp EEG is an important clinical tool for the investigation of patients with epilepsy as it can help localize the source of epileptic activity. However, EEG is characterized by low spatial resolution, and epileptic activity arising from deep brain structures cannot be detected. These limitations can be overcome by combining EEG with fMRI, since fMRI shows good spatial resolution and sensitivity to signals of both deep and superficial brain structures. Combined EEG-fMRI recordings allow mapping of BOLD signal changes associated with interictal epileptiform discharges (IED) detected on the scalp EEG. In the past years, EEG-fMRI studies have been used to noninvasively delineate the epileptogenic zone. This section gives an overview of the methodology of EEG-fMRI. We discuss the role of EEG-fMRI in presurgical evaluation (including MRI-negative cases) and the yield of EEG-fMRI based on postsurgical evaluation studies. Novel techniques in the field will be demonstrated
Background of EEG-fMRI
It is not trivial to record an EEG in an MRI environment. During scanning, the rapidly changing magnetic field induces a strong current which results in a high-amplitude gradient artefact in simultaneous EEG recordings. The development of gradient artefact correction algorithms has made it possible to record the EEG continuously during the fMRI investigation (43). An MRI-compatible EEG system with a high sampling rate (several kHz) is needed for the recording, in order to fully capture the shape of the gradient artefact for successful artefact correction. Online correction of gradient artefacts can be applied to enable visual inspection of the EEG during the EEG-fMRI recording. In addition to gradient artefacts, many EEG data sets are affected by heartbeat-synchronous artefacts. These so-called pulse or ballistocardiographic artefacts are caused by subtle pulse-synchronous movements of the head and can be removed by different artefact correction methods (44, 45). For a more detailed description of the EEG-fMRI setup and artefact correction algorithms please see recent review articles (46, 47).
Statistical analysis
The preprocessing of fMRI images does not differ from standard fMRI analysis and includes realignment, smoothing, and normalization in the case of group analyses. Standard analysis uses the event-related general linear model-based approach in which the timing of events (e.g., IED) is used to build time series for the statistical analysis. In the standard analysis, the timing of the events is convolved with the standard hemodynamic response function which peaks approximately 5 seconds after the event. Statistical maps show voxels significantly correlated with the marked event in the EEG. However, the shape and latency of the hemodynamic response function (HRF) might vary with age or different brain regions, or show altered response in epilepsy. If a more flexible HRF is applied, the sensitivity of EEG-fMRI results can be improved. In addition to the standard HRF, a more variable shape of the HRF can be achieved by including the derivative of the HRF (48) by estimating noncanonical HRFs (49, 50) or using a set of different HRFs (51).
EEG-fMRI in focal epilepsy
The EEG-fMRI was developed as a tool which might have clinical implications in presurgical evaluation by helping to localize the brain areas presumably generating IED. Early studies showed IED-associated BOLD signal changes concordant with electroclinical data in approximately 50% of the patients (52, 53). Improvements of the statistical model by using multiple HRFs or fitting individual HRFs could increase the sensitivity of BOLD signal detection (51–54). The shape of the HRF might differ, especially in children; and deactivations seem to occur more frequently in children with focal lesional epilepsy than activations in the epileptogenic zone compared to adults (55, 56). Modeling spike-related BOLD signal changes in children might be even more complex, since BOLD signal changes which precede the spike can be found in pediatric patients (57). Increasing the field strength (3 Tesla versus 1.5 Tesla) also improves the sensitivity of BOLD signal detection (58, 59). Patients selected for EEG-fMRI studies should have frequent IED to capture a sufficient number of IED during the EEG-fMRI investigation. However, often none or very few IED is recorded which leads to insufficient statistical power in the analysis and consequently to inconclusive results. To overcome this problem, Grouiller and coworkers applied a voltage map-based analysis in which they built scalp voltage maps of averaged IED recorded during long-term clinical monitoring and computed the correlation of this map with the EEG recorded inside the scanner. In patients with previously inconclusive studies, BOLD changes concordant with intracranial EEG or the resection area were detected. Even in patients in whom no clear IED in the scanner EEG were detected, this voltage map-based analysis yielded conclusive results (60). The sensitivity of the detection of IED-related BOLD signal changes can be further increased, if additional EEG features, such as sleep specific activity, are modeled (61).
IED-associated BOLD signal and the epileptogenic zone
The relationship between IED-associated BOLD signal and the epileptogenic zone was investigated by comparing BOLD signal changes to intracranial EEG recordings performed after the EEG-fMRI investigation: Bénar and colleagues were able to show that active electrodes in the intracranial EEG recordings were close to the areas of IED-associated BOLD response (62). Good concordance between IED-associated BOLD signal changes and seizure onset determined by intracranial EEG after the EEG-fMRI investigation was also demonstrated in several cases in EEG-fMRI studies of focal epilepsy (51, 63, 64). The studies mentioned above compared EEG-fMRI results with intracranial EEG recorded on different days. Recently, safety studies made it possible to perform an EEG-fMRI recording in patients with intracranial electrodes simultaneously (icEEG-fMRI) (65, 66). In two patients investigated by Vulliemoz and colleagues no IED was detected in scalp EEG-fMRI recordings performed prior to the implantation, while icEEG-fMRI revealed clear IED in these patients. Until now, only few patients with icEEG-fMRI have been reported. These studies showed BOLD response close to the electrodes from which IED were recorded (67, 68). However, BOLD signal changes, not only in the presumed epileptogenic zone but also in distant areas, were frequently detected in both icEEG-fMRI and scalp EEG-fMRI studies (67–71). These distant BOLD signal changes may reflect remote effects of the epileptic IED and could be explained in part by propagated epileptic activity (72, 73).
Most EEG-fMRI studies show both positive and negative BOLD responses. Positive BOLD responses result from increased neuronal activity compared to baseline (74), while negative BOLD responses might reflect suppressed neuronal activity (75). The IED-related negative BOLD signal changes might be caused by remote inhibition (76).
EEG-fMRI as a nonivasive tool in presurgical evaluation
Zijlmans and colleagues evaluated the role of EEG-fMRI in the preoperative work-up of patients with medically refractory epilepsy (77). The authors investigated 29 patients with EEG-fMRI who were denied surgery due to an unclear seizure focus. Only positive BOLD signal changes that were topographically related to the IED field were considered. Eight patients fulfilled this criterion and were re-evaluated for surgery based on the EEG-fMRI results. Four of these patients did not show any structural abnormalities. In four patients, including one MRI-negative case, EEG-fMRI improved identification of the seizure focus, whereas EEG-fMRI showed either mulitfocality or widespread BOLD response in the remaining four patients.
Most EEG-fMRI studies included both lesional and MRI-negative cases. We specifically addressed the yield of EEG-fMRI in patients with MRI-negative frontal lobe epilepsy. These patients are usually not considered good candidates for surgery, since delineation of the epileptogenic zone is difficult due to widespread interictal discharges, rapid spreading of ictal EEG changes, large areas which are inaccessible to scalp EEG, a large variety of seizure semiology, and low sensitivity of PET and SPECT studies. The aim of this study was to investigate whether EEG-fMRI can add meaningful information about the epileptic focus in the presurgical evaluation of patients with MRI-negative frontal lobe epilepsy. Good concordance between positive BOLD signal changes and postoperative pathological analysis or other imaging modalities was found in eight out of nine patients (78). An example of the comparison between EEG-fMRI results and methods of the presurgical evaluation in a patient with focal epilepsy is depicted in Figure 8.1. A postsurgical study showed that surgical resection which included the areas of positive BOLD response was associated with a good postsurgical outcome (79). The EEG-fMRI was recorded in ten patients undergoing presurgical evaluation; the locations of preoperative, IED-associated BOLD signal changes were compared with the resected area and postoperative outcome. Seven of ten patients were seizure-free following surgery and the area of maximum BOLD signal change was found within the resected area in six of seven patients. In the remaining three patients with only reduced postsurgical seizure frequency, areas of significant IED-correlated BOLD signal change lay outside the resection. Another study by Thornton and colleagues investigated patients with focal cortical dysplasia and compared IED-associated BOLD signal changes with the seizure onset zone (SOZ) based on icEEG recording and postoperative outcome 1 year after surgery (80). Eleven of 12 patients had significant IED-related hemodynamic changes. The fMRI results were concordant with the SOZ in five of 11 patients, all of whom had a solitary SOZ on icEEG. Four of five had > 50% reduction in seizure frequency following resective surgery. Two of these patients showed normal structural MRI. Another patient with normal structural MRI, who had a solitary SOZ on icEEG and concordant BOLD signal changes, was treated with gamma knife surgery and had a poor outcome. The remaining six of 11 patients had widespread or discordant regions of IED-related fMRI signal change. Five of six had either a poor surgical outcome (< 50% reduction in seizure frequency) or widespread SOZ precluding surgery. The authors concluded that EEG-fMRI provides useful additional information about the SOZ in patients with focal cortical dysplasia: widely distributed discordant regions of IED-related hemodynamic change appear to be associated with a broad SOZ and poor postsurgical outcome.
Figure 8.1 Comparison between EEG-fMRI and methods of the presurgical evaluation in a patient with MRI-negative frontal lobe epilepsy. (A) interictal EEG (average montage): focus F4; (B) interictal fMRI: positive BOLD response frontopolar; no negative BOLD response; (C) ictal EEG: rhythmic spike and wave F4; (D) ictal fMRI: positive BOLD response frontopolar, negative BOLD response frontal and posterior cingulated; (E) MRI: suspicious, deep right-middle frontal sulcus; (F) ictal SPECT: hyperperfusion frontal right; (G) FDG-PET hypometabolism frontal right; (H): postoperative MRI.
If a method is used for clinical purposes, it has to show good reproducibility. Gholipour and colleagues demonstrated reproducible EEG-fMRI results, supporting that this technique might be used for clinical purposes. Their findings indicate that the sensitivity of EEG-fMRI scans could be increased by scanning at 3T rather than at 1.5T (58). However, what can EEG-fMRI tell us that EEG cannot? Pittau and coworkers evaluated the new localizing information generated by EEG-fMRI compared to traditional EEG and demonstrated additional information in many patients. Eleven of 33 patients studied were MRI-negative cases. In nine of these cases the BOLD signal changes were concordant with the spike EEG field; in six of these cases the BOLD signal changes provided additional information to the scalp EEG (59). An example of a patient with MRI-negative epilepsy is depicted in Figure 8.2. It is important that results are interpreted carefully, since EEG-fMRI does not only show areas of the presumed epileptogenic zone, but also areas (also distant areas) that might be indirectly influenced by the IED. If EEG-fMRI results are considered in the context of other investigations of presurgical evaluation, they can contribute to a better understanding of the epileptic zone in a specific patient.
Figure 8.2 Patient with MRI-negative frontal lobe epilepsy. The marked events were Fp2–F8 spikes. The BOLD response showed limited activation in the lateral right orbitofrontal region. The patient underwent a depth electrode study. Five electrodes were inserted into the right hemisphere: one in the orbitofrontal region (OF), one aiming for the amygdala (A), one in the anterior hippocampus (H), one in the mid-insula (IM), and one in the posterior hippocampus and parahippocampus (PH). The black points and lines indicate the electrodes visible in these views and are obtained by coregistration with the BOLD map. The intracranial study revealed a very active epileptic generator in the lateral portion of the right orbitofrontal lobe (ROF6– ROF10). A limited right frontal corticectomy was performed, and histology showed focal cortical dysplasia type I. Top: scalp EEG, bipolar montage, Fp2–F8 spikes. Bottom: intracerebral stereo EEG. Arrow indicates the interictal event thought to correspond to the scalp spike.
Seizures
Seizures cannot be predicted and are only rarely recorded during the short time of an EEG-fMRI. Analyzing a seizure is even more difficult, as it is often accompanied by extensive movements which can make the analysis of the data impossible. To avoid seizure-induced motion problems, Federico and colleagues analyzed the BOLD signal prior to the beginning of a clinical seizure in three patients with focal epilepsy and found BOLD signal changes in the preictal state (81). Studies in short seizures without seizure-induced movements showed extensive seizure-associated BOLD signal changes, which also included the presumed SOZ (82, 83). A study by Tyvaert and colleagues on malformations of cortical development demonstrated different BOLD signal changes for interictal and ictal events (64). While the above-mentioned studies were analyzed in a block design, new analysis techniques allow investigation of the dynamics of seizure-associated BOLD signal. The dynamic analysis of seizures can reveal regions of seizure onset and propagation (84–86). Tyvaert and colleagues studied ten patients with seizures inside the scanner; three of the patients did not show structural abnormalities in the MRI. In nine patients, including all MRI-negative cases, the location of the first activation of the dynamical analysis coincided with the estimated focus based on seizure semiology, interictal and scalp EEG, and intracranial EEG (if available).
Novel techniques
The EEG-fMRI localizes epileptic foci by detecting BOLD signal changes associated with epileptic events visible in the EEG. However, scalp EEG is insensitive to activity restricted to deep structures, and recording the EEG in the scanner is complex. A recent study showed that it might be possible to detect epileptic activity from the fMRI data without the help of an EEG. Based on a wavelet model of the fMRI data (2D-temporal cluster analysis), Lopes and colleagues were able to detect similar results compared to an EEG-based fMRI analysis (87). The method is based on the assumption that the resting brain does not show significant BOLD variations in the HRF other than those associated with IED in the epileptic brain. The method could provide results in patients in whom IED on the scalp are not visible, and could allow the identification of IED epileptic networks in fMRI without EEG. The sensitivity of EEG-fMRI studies could be further increased by new fast MRI sequences. In such fMRI sequences, called magnetic resonance–encephalography (MREG), fMRI images are acquired with a temporal resolution of 100 ms (88). Since 20–30 times more images after each IED are recorded than during a classical fMRI sequence, the statistical power of the study increases. In a first study of 13 patients with focal epilepsy the average t-value of BOLD responses was significantly higher in the MREG than in the standard fMRI sequence with a temporal resolution of 2.6 s. Moreover, BOLD responses with MREG were found in patients in whom the standard fMRI did not show any BOLD changes. The BOLD signal changes were even detected in single spike analyses (89–90). The high temporal resolution might allow the differentiation between onset and propagation of epileptic activity.
However, not only IED-associated EEG-fMRI might play a role in the presurgical evaluation. Functional connectivity studies measure how different brain areas are connected during the resting state of the brain. Negishi and colleagues proposed functional connectivity as a predictor of epilepsy surgery outcome (91). The IED-related EEG-fMRI was performed for each patient. An activation cluster that overlapped most with the planned resection area was chosen, in order to perform a functional connectivity analysis. Patients who had a poor postsurgical outcome showed less lateralized functional connectivity than patients who were seizure-free after the surgery.
Conclusions on EEG-fMRI
There are few studies that specifically address the value of EEG-fMRI in MRI-negative refractory epilepsy. Most studies investigated both lesional and nonlesional cases. These studies show that IED and seizure-related EEG-fMRI can be used to noninvasively detect the epileptogenic zone. It is of note that EEG-fMRI is only an additional tool in the presurgical work-up, and the results have to be considered in the context of other investigations of the presurgical evaluation. Postsurgical studies suggest that surgical resection that includes the areas of positive BOLD response, and a lateralized functional connectivity, is associated with a good postsurgical outcome. Novel techniques such as fast MRI sequences may further increase the value of EEG-fMRI.
References




















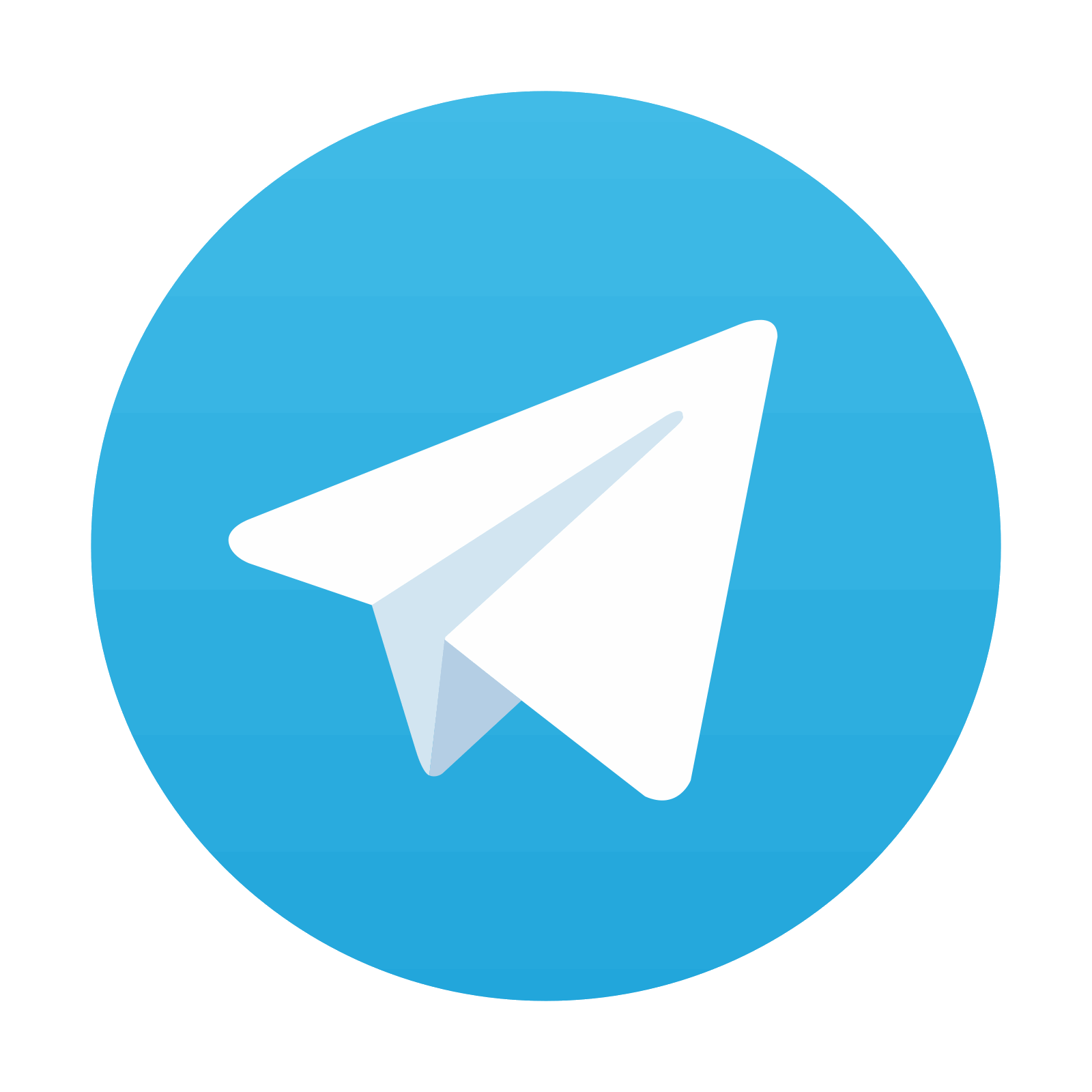
Stay updated, free articles. Join our Telegram channel
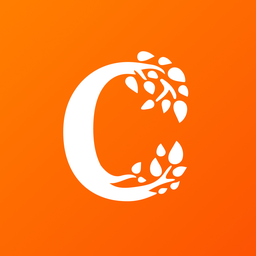
Full access? Get Clinical Tree
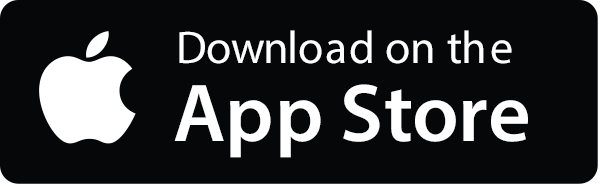
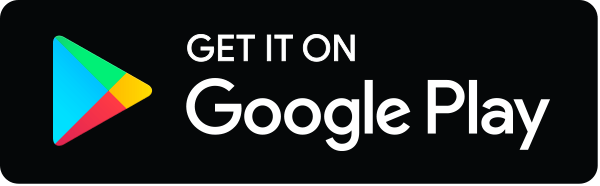
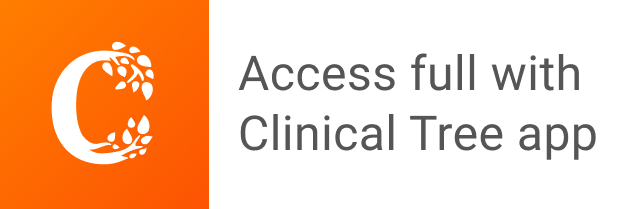