Chronobiology and Circadian Rhythms
Arthur Andrews
Selim Benbadis
INTRODUCTION
The rotation of the Earth on its axis over a 24-hour period produces the well-known changes in the physical environment experienced by its inhabitants. The resultant light-dark cycle and the demands it imposes has produced evolutionary change in all forms of life since the beginning of time. The past several decades of investigation have demonstrated that the 24-hour nature of life is not simply a result of these external cues, but, indeed, due to an internal time-keeping system in the brain (1).
Sleep across species occurs within rigidly defined times of day and night. The 24-hour or circadian biochemical, physiologic, and behavioral rhythms are regulated by a biologic clock. The regularly occurring variation in these parameters has analogs in all eukaryotic species, including single-celled algae (2). The suprachiasmatic nucleus (SCN) located in the hypothalamus has been thought to be the source of this rhythmicity (3) (Fig. 6-1). Sleep is regulated by the dual interaction of circadian and homeostatic processes. The individual’s state of wakefulness or sleep at any moment in time is dependent upon the interaction between sleep debt and the circadian clock (4). Many diverse species share common genetic and molecular elements in regard to circadian systems.
Indeed, the awareness of sleep disorders as a major health concern has increased dramatically over the last decade. The number of sleep disorder centers in the United States has doubled since 1996 (4). Sleep disorders and the treatment of these disorders have significant socioeconomic consequences.
CIRCADIAN RHYTHMS VERSUS SLEEP-WAKE RHYTHMS
Circadian Rhythms
Reproducible changes of essentially all physiologic and behavioral variables occur over a 24-hour period (5). Circadian rhythms are the manifestation of an endogenous oscillatory signal with a near-24-hour period that occurs in synchrony with the 24-hour periodicities in the physical environment. The aforementioned endogenous oscillator is termed the circadian clock. The internal milieu of the organism is also organized so that internal changes crucial to health are coordinated. The internal clock must adjust its period each cycle to compensate for differences between its intrinsic periods and the 24-hour period of the environmental day and be synchronized in order for rhythms to occur at appropriate phases (3). This process is known as entrainment. Adaptation and survival mandate that the circadian clock be synchronized and entrained by environmental cues.
Sleep-Wake Rhythms
Many rhythms are dependent on the sleep-wake state of the organism and not primarily the circadian timekeeper. An animal placed in an environment where all possible 24-hour external cues are eliminated will continue to demonstrate diurnal rhythms. The period is approximately 24 hours and is called circadian (1). The sleep-wake cycle is one such rhythm. The human sleep-wake cycle is not simply driven by the circadian pacemaker, but instead is generated through interactions of circadian rhythmicity,
a sleep-wake oscillatory process, circadian photoreception, and feedback from the sleep-wake cycle onto these processes (6). As one can infer, the interplay of influence from the sleep-wake system and the circadian system manifests as the expression of physiologic, behavioral, and biochemical processes of the organism. Both waking performance and sleep consolidation are contributed to equally by these two systems (6). The sleep-wake oscillator acts as a homeostat and regulates the level of sleep debt. As the normal day progresses, the SCN generates an arousal signal that increases in strength. This signal begins to decline by 10 PM, with nadir at 6 AM. It is the presence of this arousal signal that produces the monophasic sleep-wake cycle and maintains its timing. Sustained wakefulness leads to increased sleep need. This requirement for sleep is opposed by the circadian pacemaker. Rapid eye movement (REM) sleep is primarily under the control of circadian rhythmicity and is normally at its peak near the end of the sleep period. Slow-wave sleep timing primarily reflects sleep-wake homeostasis (7). Interestingly, there is also evidence that the SCN also promotes sleep. The SCN clock is also responsible for the endogenous regulation of moment-to-moment waking behavior as measured by fatigue, alertness, and cognitive performance (3).
a sleep-wake oscillatory process, circadian photoreception, and feedback from the sleep-wake cycle onto these processes (6). As one can infer, the interplay of influence from the sleep-wake system and the circadian system manifests as the expression of physiologic, behavioral, and biochemical processes of the organism. Both waking performance and sleep consolidation are contributed to equally by these two systems (6). The sleep-wake oscillator acts as a homeostat and regulates the level of sleep debt. As the normal day progresses, the SCN generates an arousal signal that increases in strength. This signal begins to decline by 10 PM, with nadir at 6 AM. It is the presence of this arousal signal that produces the monophasic sleep-wake cycle and maintains its timing. Sustained wakefulness leads to increased sleep need. This requirement for sleep is opposed by the circadian pacemaker. Rapid eye movement (REM) sleep is primarily under the control of circadian rhythmicity and is normally at its peak near the end of the sleep period. Slow-wave sleep timing primarily reflects sleep-wake homeostasis (7). Interestingly, there is also evidence that the SCN also promotes sleep. The SCN clock is also responsible for the endogenous regulation of moment-to-moment waking behavior as measured by fatigue, alertness, and cognitive performance (3).
Certain drug therapies that combine chronobiotic/hypnotic effects have been shown to shorten the time to adaptation of both circadian rhythmicity and sleep-wake homeostasis to a shift in dark/sleep cycle. Buxton et al. (7) used the benzodiazepine triazolam to successfully facilitate adaptation to an 8-hour delay shift of sleep-wake and dark-light cycles simulating westward travel.
The circadian rhythms of alertness and performance efficiency run in parallel to body temperature. Alertness is the ability to maintain attention. The ability to successfully complete tasks as varied as working memory, complex executive functions, and logical reasoning is termed performance (3). The controlled laboratory setting is helpful in eliminating the differences that can be obtained in peak performance as a result of level of task. The nadir of alertness and performance typically occurs early in the
morning at 4 to 6 AM at the time of the low point in core body temperature (8). An individual’s subjective report of an insufficient amount of energy to complete a task is considered fatigue (Fig. 6-2).
morning at 4 to 6 AM at the time of the low point in core body temperature (8). An individual’s subjective report of an insufficient amount of energy to complete a task is considered fatigue (Fig. 6-2).
ANATOMY AND PHYSIOLOGY OF THE HUMAN (MAMMALIAN) CIRCADIAN SYSTEM
Nearly 30 years ago, the paired SCN of the anterior hypothalamus was found to be the pacemaker for mammalian circadian rhythms. Animal lesion studies were the primary means to this discovery. The metabolic activity of the SCN has been shown to peak during the subjective day for both nocturnal and diurnal animals (3). Individual SCN neurons have intrinsic 24-hour oscillatory properties in culture. In vivo, these neurons generate a coordinated circadian signal to the remainder of the brain and the peripheral organs (1). One study (9) demonstrated that transplantation of SCN-containing fetal neural tissue into arrhythmic hamsters restored circadian locomotor activity.
SCN neurons are very densely packed. Animal anatomic studies suggest that there is a dorsomedial and ventrolateral subdivision (10). Neurochemicals in the ventral SCN respond to photic stimuli, whereas neurochemicals synthesized in the dorsal SCN demonstrate endogenous rhythms but do not respond to changes in light (3).
An environmental synchronizing source is required to entrain the SCN circadian clock to the 24-hour day. Indeed,
the light-dark cycle serves this purpose in the majority of mammals. Pulses of light effect increases or decreases in the firing rates of specialized neurons in the SCN (11).
the light-dark cycle serves this purpose in the majority of mammals. Pulses of light effect increases or decreases in the firing rates of specialized neurons in the SCN (11).
The retinohypothalamic tract (RHT) is a direct projection from the retinal ganglion cells to the SCN. Photic input from the RHT is mediated by an excitatory amino acid, such as glutamate.
Neuropeptide Y, monoamines, gamma-aminobutyrate (GABA), and acetylcholine also appear to mediate important projections to the clock; pharmacologic manipulation of those systems can impact circadian phase and amplitude (2). Additional areas of the brain also provide input to the SCN. These include the thalamus, brainstem, and certain forebrain areas. Nonphotic entrainment on the SCN is thought to be accomplished via serotonin and geniculate-hypothalamic tract (GHT) pathways. Neuropeptide Y is the neurotransmitter of the GHT. Serotonergic (5HT) projections from the brainstem raphe nuclei project directly and indirectly. These projections are now considered to be important in the circadian effects of nonphotic stimuli. Nonphotic stimuli can induce increased arousal, and therefore, changes in behavior that can influence the phase and period of circadian rhythms in certain animals (11).
Studies in the rat have found several pathways from the SCN to arousal and sleep centers. Adrenergic (locus caeruleus), serotonergic (dorsal raphe), histaminergic (tuberomammillary nucleus), and orexin systems receive projections from the SCN (Fig. 6-3). By using immunohistochemical techniques and retrograde transport of fluorescent dyes. Four major efferents from the SCN were identified. These projections include (a) fibers to the optic tracts terminating in the ventral lateral geniculate nucleus; (b) a group of fibers to the anteroventral periventricular nucleus, medial preoptic area, lateral septum, bed nucleus of the stria terminalis, parataenial nucleus, and parts of the paraventricular nucleus of the thalamus; (c) ventral to the paraventricular nucleus of the hypothalamus; and (d) anterior hypothalamic area, retrochiasmatic area, lateral hypothalamus, and ventromedial hypothalamus (3).
MOLECULAR GENETICS OF CIRCADIAN RHYTHMS
The major source of the generation of the circadian rhythm is within the neurons of the SCN. A feedback cycle involving the various transcribed gene products is the driving force of the oscillatory machinery. The organism is, therefore, able to prepare itself for the regular environmental changes.
Period (Per), the first gene that encodes a clock component, was found in the fruit fly Drosophila in 1971 (3). Transgenic flies having a heat-inducible copy of Per demonstrate long-lasting phase shifts in locomotor activity circadian rhythm when subjected to temperature pulses (13). In addition, the lack of a Per gene or a missense mutation of Per has been shown to cause lack of locomotor activity and shortening/lengthening of the free-running period of the locomotor rhythm, respectively. Timeless (Tim) is an additional oscillatory component in Drosophila.
Tim may be important in regulating the entry of Per into the nucleus. In Drosophila lacking Tim, the loss of behavioral circadian rhythms is associated with loss of daily fluctuations of Per mRNA (14). The Tim:Per complex suppresses the transcription of Tim and Per.
The molecular components of the circadian Clock gene in Drosophila and the filamentous fungi Neurospora were identified in the 1980s (1). In 1997, the first mammalian circadian clock gene Clock was identified and sequenced. Mice with homozygous mutations in the Clock gene demonstrate progressive lengthening of circadian rhythms and eventually become arrhythmic in constant darkness (15).
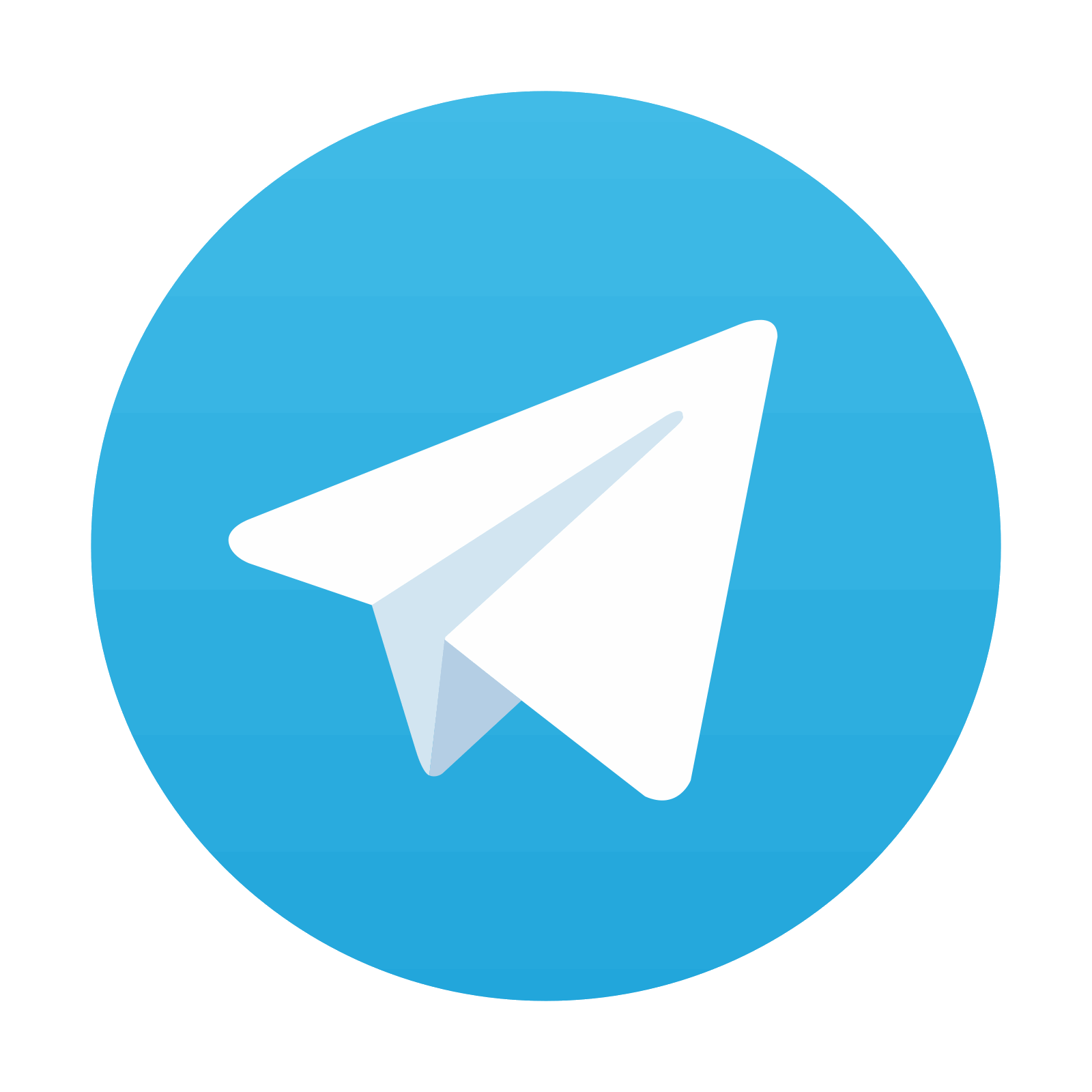
Stay updated, free articles. Join our Telegram channel
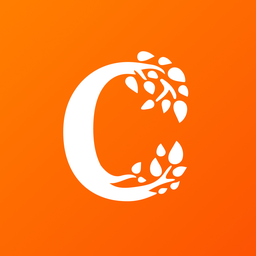
Full access? Get Clinical Tree
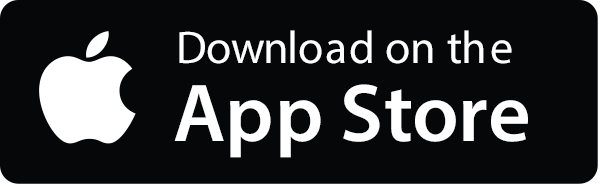
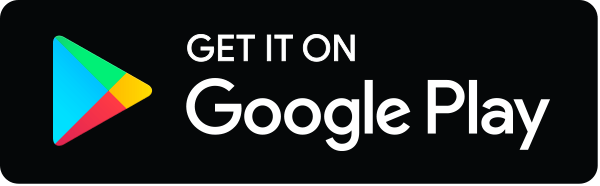