Noninvasive neuroimaging aids in surgical planning and in counseling patients about possible risks of surgery. Magnetoencephalography (MEG) performs the most common types of surgical planning that the neurosurgeon faces, including localization of epileptic discharges, determination of the hemispheric dominance of verbal processing, and the ability to locate eloquent cortex. MEG is most useful when it is combined with structural imaging, most commonly with structural magnetic resonance (MR) imaging and MR diffusion imaging. This article reviews the history of clinical MEG, introduces the basic concepts about the biophysics of MEG, and outlines the basic neurosurgical applications of MEG.
For presurgical evaluation of resection of a mass or other lesion, noninvasive neuroimaging aids in the surgical planning and in counseling patients about possible risks of the surgery. Magnetoencephalography (MEG) performs the most common types of surgical planning that the neurosurgeon faces, including localization of epileptic discharges, determination of the hemispheric dominance of verbal processing, and the ability to locate eloquent cortex. MEG is most useful when it is combined with structural imaging, most commonly combined with structural magnetic resonance imaging (MRI) and MR diffusion imaging. This article reviews the history of clinical MEG, introduces the basic concepts about the biophysics of MEG, and outlines the basic neurosurgical applications of MEG.
Brief history of MEG
In 1968, David Cohen ( Fig. 1 ) used a room-temperature copper coil as a detector to record the first magnetoencephalogram at the University of Illinois. Later, at the Massachusetts Institute of Technology, he built a more elaborate shielded room. At about the same time, James Zimmerman and colleagues developed the Superconducting Quantum Interference Device (SQUID), which uses the Josephson junction to measure tiny magnetic fields. SQUID requires cooling to liquid helium temperatures, and has a sensitivity of several hundreds of times that of a copper coil. Zimmerman brought this detector to Cohen’s room, and this combination of shielding and detector allowed the first clear measurements of the body’s magnetic fields. After they measured the heart, Cohen next recorded the first MEG measured with a SQUID.

For the initial measurements of magnetic brain activity, physicists and neuroscientists used a single or a just few magnetometers. Localizing activity with such a low number of sensors required moving them to maintain sampling from various locations over the head. This process was time consuming and not practical for routine clinical use. Clinical MEG became possible when MEG systems were developed that provided coverage of approximately 7 to 12 cm, enough to produce a field map large enough to visualize the magnetic field lines from a single dipole source; this made it possible to localize the activity in a registered brain MRI.
Biophysical principles of magnetoencephalography
Modern MEG systems now provide hundreds of channels that can provide whole-head coverage ( Fig. 2 ). This coverage makes it possible to map activity throughout the cerebral cortex and is critical for presurgical mapping of language areas, and for detecting propagating or widespread epileptic activity. Due to interference from extraneous magnetic fields, all MEG measurements must be performed in a magnetically shielded room, which typically consists of 2 to 4 layers of aluminum and multiple layers of ferromagnetic shielding.
Unlike other hemodynamic techniques (functional MRI [fMRI] and positron emission tomography [PET]), both MEG and electroencephalography (EEG) directly measure electric brain activity. The neural generators of the MEG and EEG are identical. There are critical differences that make them both useful and complementary. MEG preferentially detects activity in superficial, nonradial areas of cortex, such as the fissural cortex of the cerebral hemispheres; this is particularly advantageous if the area of interest is also radial, such as the primary somatosensory cortex, which lies in the walls of the sulci.
Much of the neural activity measured by MEG originates as the postsynaptic activity in the pyramidal cells of the cerebral cortex. MEG measures the vector sum of postsynaptic potentials, as contrasted with BOLD (Blood Oxygenation Level Dependent) fMRI and some form of PET imaging that reflects neural activity indirectly through changes in blood flow. MEG localizes neural activity more accurately than EEG because magnetic fields are less perturbed than electrical potentials by overlying brain structures: scalp, skull, cerebrospinal fluid, meninges, and vascular structures. Recently, statistical combination of structural MRI and fMRI with MEG has taken a great stride forward by yielding the maximum benefit from each technique into a single image.
The calculation of the magnetic field is more straightforward than that of the electric field because of the symmetries and conductivity distribution of the human head, and because the EEG also is influenced by the extracellular volume current, which is difficult to model accurately. All currents, both intracellular and extracellular, generate magnetic fields, but because of the near spherical shape of the head, one can calculate the resultant magnetic fields caused by primary currents without taking into account the conductivity layers of the head.
Biophysical principles of magnetoencephalography
Modern MEG systems now provide hundreds of channels that can provide whole-head coverage ( Fig. 2 ). This coverage makes it possible to map activity throughout the cerebral cortex and is critical for presurgical mapping of language areas, and for detecting propagating or widespread epileptic activity. Due to interference from extraneous magnetic fields, all MEG measurements must be performed in a magnetically shielded room, which typically consists of 2 to 4 layers of aluminum and multiple layers of ferromagnetic shielding.
Unlike other hemodynamic techniques (functional MRI [fMRI] and positron emission tomography [PET]), both MEG and electroencephalography (EEG) directly measure electric brain activity. The neural generators of the MEG and EEG are identical. There are critical differences that make them both useful and complementary. MEG preferentially detects activity in superficial, nonradial areas of cortex, such as the fissural cortex of the cerebral hemispheres; this is particularly advantageous if the area of interest is also radial, such as the primary somatosensory cortex, which lies in the walls of the sulci.
Much of the neural activity measured by MEG originates as the postsynaptic activity in the pyramidal cells of the cerebral cortex. MEG measures the vector sum of postsynaptic potentials, as contrasted with BOLD (Blood Oxygenation Level Dependent) fMRI and some form of PET imaging that reflects neural activity indirectly through changes in blood flow. MEG localizes neural activity more accurately than EEG because magnetic fields are less perturbed than electrical potentials by overlying brain structures: scalp, skull, cerebrospinal fluid, meninges, and vascular structures. Recently, statistical combination of structural MRI and fMRI with MEG has taken a great stride forward by yielding the maximum benefit from each technique into a single image.
The calculation of the magnetic field is more straightforward than that of the electric field because of the symmetries and conductivity distribution of the human head, and because the EEG also is influenced by the extracellular volume current, which is difficult to model accurately. All currents, both intracellular and extracellular, generate magnetic fields, but because of the near spherical shape of the head, one can calculate the resultant magnetic fields caused by primary currents without taking into account the conductivity layers of the head.
Interpretation of MEG
Intelligent interpretation of MEG requires the examination of the waveforms recorded by the SQUID sensors, followed by a source estimate that reflects the site of activity in the brain. The raw measurement of MEG is a time-varying magnetic field. For epilepsy discharge mapping, segments of the activity are used for source analysis, often without any signal averaging. To improve the signal-to-noise ratio of measured signals for evoked activity, such as language laterality, it is often necessary to average several (typically around 100 trials) responses from identical or similar stimuli. The signal is typically averaged based on the timing of the presented stimulus, the subject’s response, or the peak of activity, such as the peak of an epileptic spike. Averaging lowers the effects of extraneous activity, effectively improving the overall signal-to-noise ratio of the desired activity.
Source Modeling
Source modeling determines the origin of the measured neuromagnetic fields, which is the goal of most MEG measurements. The mathematics behind this analysis is known as the inverse problem. In general, the inverse solution of electromagnetic measurements is nonunique and ill-posed. If proper assumptions are made, however, the solution becomes solvable. Some of most commonly used source analysis methods used clinically are discussed here.
Equivalent current dipole
To make the inverse solution tractable, one can approximate that the activity from primary sensory or motor cortex originates as a single equivalent current dipole (ECD). Physiologically this is plausible, given that a limited patch of cortex is synchronously activated and that the sensors are at least a few centimeters from the source. The ECD provides spatial information, magnitude (current dipole moment), and direction. The ECD is typically computed using a standard iterative least-square algorithm, which can also provide a measure of dipole parameter confidence, as well as the best-fitting parameters such as goodness-of-fit measure.
Thus, by assuming a single source, the inverse problem has a unique solution, which works particularly well for primary sensory areas, focal epilepsies, and higher cognitive areas that have a focal source. Further advances in the ECD approach, for both EEG and MEG, have made it possible to find multiple ECDs with a multidipole approach, such as that developed by Hari and colleagues for somatosensory activation. One approach to investigate temporal changes in different areas of the brain is known as the time-varying dipole model. In this model, a series of dipoles are modeled such that the locations are fixed, but allowing the amplitudes to vary over time ( Fig. 3 ). This ECD approach works well for sequentially or simultaneously activated cortical sources, although the fine spatial details are lost because the measurements are obtained at least 3 cm from the brain sources.
Distributed solutions
Minimum norm estimate
If a large patch of cortex is activated, or if there are several areas of cortex activity simultaneously, the single ECD solution may be misleading. In practice this may be suspected when a dipole localizes too deep to be physiologically plausible, that is, in the deep white matter. In such cases, distributed solutions such as the minimum norm estimate (MNE), the minimum current estimate, or a beamformer solution may be more accurate. Although numerous other inverse solutions exist, this review concentrates on the MNE and beamformer solutions.
Originally pioneered by Hämäläinen and Ilmoniemi, and improved on by Dale and colleagues, the MNE is now available in various software packages, from freeware to commercially available software. The MNE does have some important limitations that must be kept in mind especially when used clinically: a depth bias and a difficulty in determining the extent of activation. In its most elementary expression, the source variance is assumed to be equal throughout the volume, and the MNE solution is biased toward the most superficial currents. One approach to lessen this effect is to use a cortical constraint obtained from the anatomic MRIs. In addition to a depth bias, determining the extent of the sources is also problematic. Simulations show that the point-spread function of estimates is a function of location. Further, the spread depends on the assumed source variance.
To compensate for the superficial bias of MNE current sources, Dale and Halgren have suggested producing a dynamic statistical parametric map (dSPM) by normalizing the estimate MNE by the signal noise. Using the dSPM, the point-spread function is more uniform across the brain and removes the superficial bias (see Fig. 3 ). In addition, the dSPM is F-distributed, allowing it to be used for hypothesis testing.
Beamformer
Another distributed source solution is commonly termed the beamformer, which is based on the principle of spatial filters. Beamformers typically are applied without a cortical constraint, although they can be modified to do so. Synthetic-aperture magnetometry (SAM) is a beamformer approach that can be applied to both raw data and averaged (evoked) MEG or EEG. More sophisticated methods, such as adaptive spatial filtering and dynamic imaging of coherent sources, use a spectral analysis with coherence or other correlations that make it possible to measure functional connectivity in the brain within a particular spectral band. This method makes it possible to localize changes in spectral power or functional connectivity that can be used to make evoked (such as language and motor mapping) or resting state functional connectivity.
Eloquent cortex evaluation
Current Presurgical Methods to Evaluate Eloquent Cortex
In patients with epilepsy or brain masses, lateralization and localization of language functioning may be critical for preserving the quality of life. At present, there are several methods to evaluate eloquent cortex, including neuropsychological testing, fMRI, MEG, the intracarotid amobarbital procedure (IAP), and invasive electrode mapping. Precise localization of the motor cortex and somatosensory cortex (central sulcus) is commonly required for frontal masses or for frontal lobe epilepsy. Verbal memory and language are the most common functions to be affected by a left anterior temporal lobectomy (ATL), a common surgery for medial temporal lobe epilepsy. Testing whether a temporal lobe is dominant for verbal function is often referred to as simply determining language lateralization (or verbal memory lateralization). Language cortex, typically the posterior language cortex, also needs to be precisely localized when a lesion is located in the posterior temporal lobe. To better understand how MEG assesses the surgical patient, the author briefly discusses the evaluation of language function.
Neuropsychological testing
Neuropsychological testing assesses the functional status of the hippocampus and predicts the neurocognitive outcome after resection of the hippocampus, but its use as a sole predictor of outcomes is limited. Neuropsychological test performance correlates with mesial temporal lobe epilepsy status and neuropsychological test performance before and after surgery, although the overall specificity is moderate. Material-specific memory loss as measured by neuropsychological test performance is observed in 20% to 30% of patients following unilateral ATL. Material-specific memory loss is far more common following left- than right-sided ATL, with 25% to 50% of left-sided ATL patients demonstrating verbal memory decline. This outcome is particularly true for patients without significant sclerosis on the surgical side. Declines in visual memory following right-sided ATL are less consistently observed but have been reported on tests that are highly spatial in nature. Accordingly, preoperative scores on material-specific memory tests have demonstrated some utility in the prediction of cognitive morbidity following anterior temporal lobectomy; however, the sensitivity of prediction is modest (56%).
Intracarotid amobarbital procedure
The IAP is used to predict language and memory outcomes following an ATL or for resections near the cortical language area. For an IAP, amobarbital is injected into the internal carotid artery through an arterial catheter, and brief neuropsychological tests are performed. The IAP generally assesses verbal memory and, to a lesser extent, memory for visual items that are not easily encoded verbally, such as scenes or faces Fig. 4 .
Limitations of the IAP
Despite its widespread use, some shortcomings to the IAP are widely known and limit its use. The IAP is invasive with a small but measurable risk of stroke, vascular damage, and infection. The results of the IAP have been shown to be unreliable in test-retest studies, which may be due to flow of anesthetic to the opposite hemisphere (transhemispheric cross-flow) and variable penetration of the vasculature to the mesial temporal lobe from the internal carotid artery. Recently there has been a shortage of amobarbital, which has also reduced the use of the procedure, at least temporarily. Pelletier and colleagues suggest 4 requirements are needed before a test could replace the IAP: (1) a high predictive power for the presence or absence of critical functions in specific brain regions; (2) a user-independent statistical methodology; (3) high spatial resolution; (4) production of reliable activation maps at the individual level. MEG meets these criteria and, in the author’s experience, its routine use has led to a reduction in the number of patients who undergo the IAP.
Language evaluation
In part due to advancements in functional neuroimaging, the IAP is being used less and less frequently. Despite the IAP being the consensus gold standard for verbal functional, it has been criticized because of potential cross-flow to the contralateral hemisphere and a typical lack of testing of territory supplied by the posterior circulation. Since the mid 1990s, MEG has been used to determine hemispheric dominance for language as well as regional language mapping of individual language areas. MEG of language areas provides an accurate noninvasive method of mapping these areas. In practice, MEG can be used to plan for an IAP and stimulation-based intraoperative mapping techniques.
Determining the location of language processing in a subject requires applying the task that best activates the desired stream of language processing. Specific language processes are complex and often include phonological, lexical, and also semantic processes. Verbal memory encoding and retrieval occurs concurrently in virtually any task, making it difficult to separate “language processing” from “memory.” Further supporting language processes are attention, motor planning (speech), and visual or auditory functions. In fMRI, semantic decision and stem completion tasks are popular as they require a response from the patient, which ensures the quality of the patient’s responses. For MEG, covert responses are more desirable, as overt (spoken) responses may lead to unacceptable motion artifacts. Nevertheless, some passive sensory paradigms requiring no patient response have been reported in the literature to be successful (see later discussion).
Hemispheric dominance for language
Determination of the language-dominant hemisphere is critical in the presurgical evaluation of patients scheduled for temporal or frontal lobe resections. For a left ATL, the determination of language laterality is done routinely with MEG and fMRI. Wada and Rasmussen determined that more than 93% of patients are left-hemisphere dominant for language, as are more than 96% of right-handed patients, although more recent studies indicate that many patients have more bilateral representation of language than the original studies. In left-handed epilepsy patients, only about 70% of patients demonstrate left hemispheric dominance for language, with about 15% of patients demonstrating bilateral language lateralization.
First proposed by Papanicolaou and colleagues, sequential dipole fitting is a robust method to determine language laterality ( Fig. 5 ). The stimuli used by Papanicolaou were both auditory and visual words. The results concur with Wada test results and electrical stimulation mapping. Szymanski and colleagues reported using simple phonetic stimuli, such as the vowel sounds /a/ and /u/, for determining the language-hemispheric dominance by summing the number of selective dipoles in the late auditory magnetic field on each hemisphere and calculating a lateralization index. Multiple groups report a strong correlation with both intraoperative mapping techniques and the results of the Wada test.
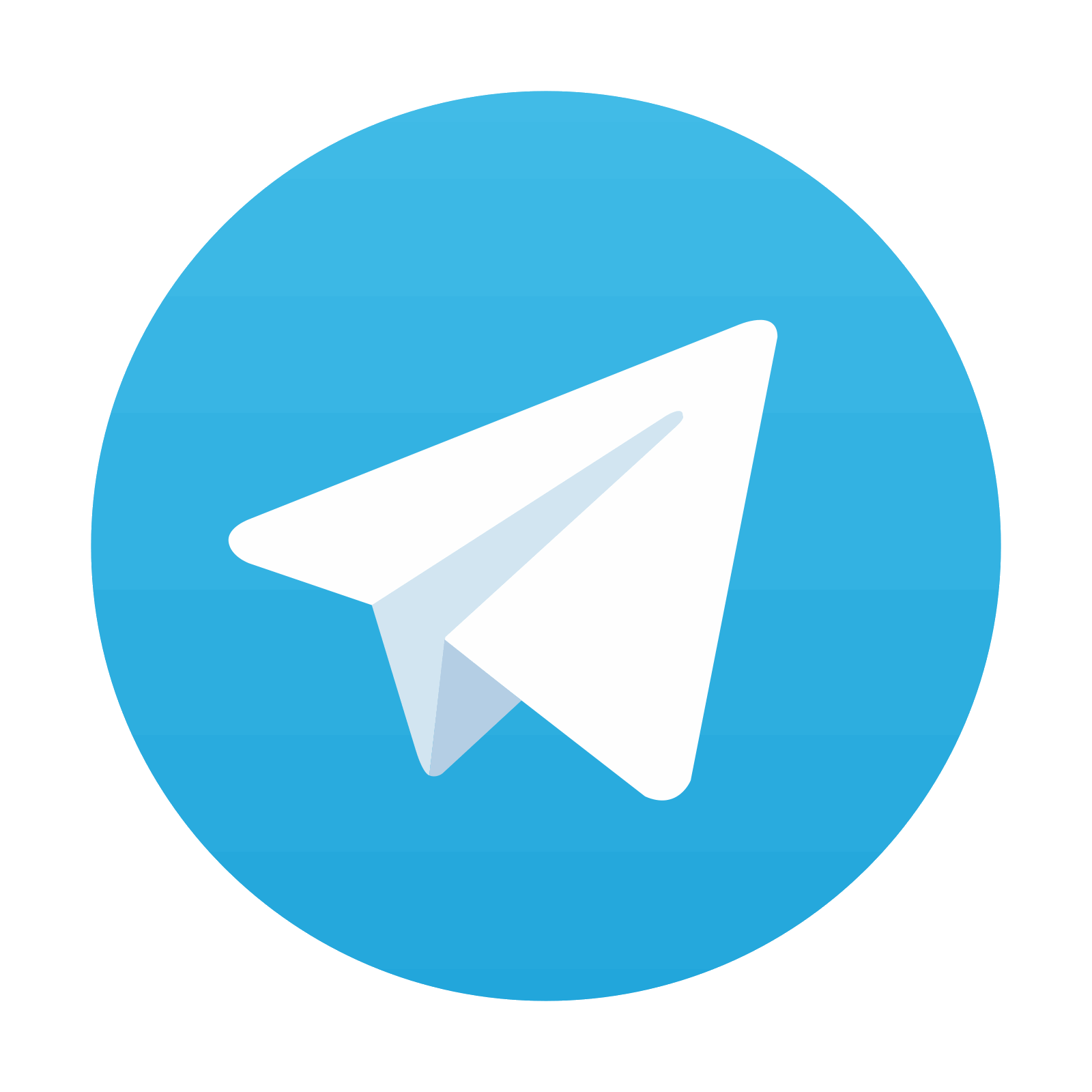
Stay updated, free articles. Join our Telegram channel
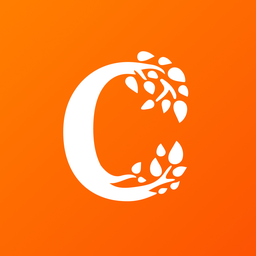
Full access? Get Clinical Tree
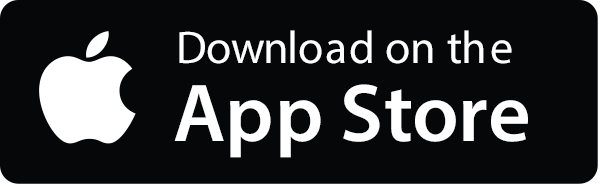
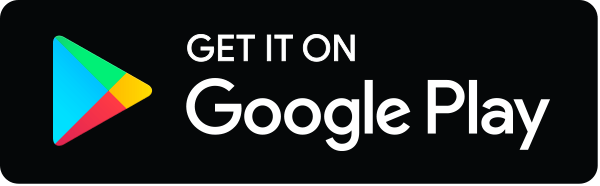
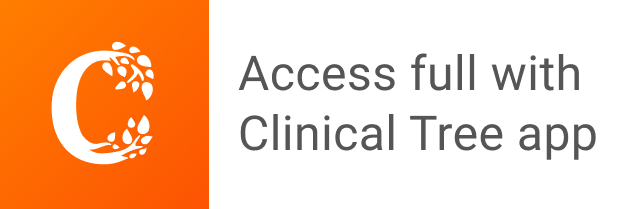