Fig. 5.1
Clinical systems neuroscience consists of multi-layered sub-disciplines with different spatial scales: molecular neuroscience (nanometer), cellular neuroscience (micrometer), systems neuroscience and cognitive/behavioral neuroscience (millimeter to centimeter). Advances in non-invasive techniques that examine brain functions have made it easier for clinicians to ask meaningful scientific questions at the systems, cognitive, and behavioral neuroscience levels. Neuroscientists, in turn, can explore a new discipline for basic research, one that holds the potential to provide solutions to unanswered questions in clinical medicine. Clinical systems neuroscience has come to the fore as a result of mutual interactions between neuroscience and clinical medicine, as distinct from unidirectional translation from basic research to clinical medicine
5.3 Clinical Systems Neuroscience: Present
Clinical systems neuroscience examines the functional neuroanatomy of the nervous systems in living humans, especially that of patients with neurological, neurosurgical, psychiatric, or other systemic diseases involving the nervous system, and does so using non-invasive methods. However, functional neurosurgery is an exception. Currently available tools such as neuroprostheses are based on electrophysiological recordings, neuroimaging, brain stimulation, and neuro-IT. The technical details and application of each method to each functional system are beyond the scope of this chapter. Hence, the chapter restricts itself to briefly summarizing the technical background and characteristics of every tool that has played a part in the emergence of clinical systems neuroscience. Likewise, discussion of every application to every neuropsychiatric disorder is beyond the scope of this chapter. Therefore, typical applications of each tool to just a few diseases are described with the objective of solving problems in clinical systems neuroscience.
5.3.1 Electrophysiology
5.3.1.1 Electroencephalography (EEG)
Since its discovery by Hans Berger in 1929, EEG has been widely used as a clinical or scientific method to monitor ongoing brain activity. Signals acquired using a scalp electroencephalogram (also abbreviated as EEG) are believed to reflect a summation of local field potentials (LFPs). LFPs recorded by EEG are considered to reflect mostly excitatory postsynaptic potentials (EPSPs) and partly inhibitory postsynaptic potentials (IPSPs) generated in the apical dendrites of pyramidal cells. Hence, an EEG mainly comprises inputs into a large population of cortical neurons which, when synchronized, may produce oscillatory EEG patterns that can be recorded from surface EEG.
A scalp EEG is recorded by means of an electroencephalograph in conjunction with electrodes attached to the scalp, often arranged according to the International 10–20 System of Electrode Placement (Fp1, Fp2, F3, F4, C3, C4, P3, P4, O1, O2, F7, F8, T3, T4, T5, T6, Fz, Cz, Pz, A1, and A2). The letters F, T, P, and O represent the frontal, temporal, parietal, and occipital lobes, respectively. The letter C refers to electrodes close to the central sulcus, corresponding to the primary motor and somatosensory cortices. The letter A refers to the earlobes. The letter z (zero) refers to electrodes placed on the midline. Even numbers and odd numbers refer to electrode positions on the right and left hemispheres, respectively. For research purposes, electrode caps with denser arrays of electrodes (e.g., 64, 128, and 256 electrodes) are available.
An EEG often reveals conspicuous oscillatory activity. Oscillatory EEG activities are classified into α (8–15 Hz), β (16–31 Hz), γ (>32 Hz), δ (<4 Hz), and θ (4–7 Hz) ranges or bands according to their frequency. Resting-state EEG activity is dominated by α-range oscillations over the parieto (P) to occipital (O) electrodes (posterior dominant rhythm) in healthy subjects. The posterior dominant rhythm is suppressed by eye opening (visual inputs) and by reduced arousal levels. Although it is unclear how posterior dominant rhythms are generated and modulated, functional MRI (fMRI) combined with EEG has started to shed light on the modulation mechanisms of the posterior dominant rhythm at rest (Omata et al. 2013). Other important rhythms are sensorimotor rhythms (SMRs), or mu rhythms; they are α-band oscillations recorded over the central (C) electrodes. SMRs are considered to reflect the idling state of the motor system since movement suppresses them. However, since SMRs are also suppressed by motor imagery or motor intention, their amplitude is now used as a feature to detect the motor intention of patients who cannot actually move (i.e., the brain–machine interface).
The normal oscillatory activities observed in EEG recording are collectively called “background EEG activity.” Diffuse slowing and/or reduction of background EEG activity indicates diffuse impairment of brain functions (diffuse encephalopathy), especially when accompanied by disturbance of consciousness. The complete loss of EEG background activity is termed “electrocerebral inactivity or silence,” which is seen in complete and irreversible brain functions like brain death. Local slowing or reduction of EEG background activity suggests focal abnormality of brain functions resulting from, for example, space-occupying lesions and ischemic lesions.
Detecting epileptiform discharges using EEG is an indispensable process in the diagnosis of epilepsy. Epileptiform discharges are distinctive waves or complexes lasting several tens of milliseconds to a few hundred milliseconds, often called “spikes” or “sharp waves,” which stand out from background activity. Physiologically, an epileptiform discharge corresponds to a paroxysmal depolarization shift (which is abnormal) and to a large EPSP occurring in a group of neurons in epileptic foci.
The advantages of scalp EEG as a tool of clinical systems neuroscience include non-invasiveness, fine temporal resolution, and prevalence in its use in research and clinical institutes all over the world. Conversely, the shortcomings of scalp EEG include poor spatial resolution/localization and inaccessibility to deep-brain structures. Poor localization can be improved by source localization techniques, which have been developed with the aid of anatomical/functional MRI (Yoshimura et al. 2012) and modeling methods (Michel et al. 2004). The development of these techniques, coupled with other visualization technology, has made EEG an even more powerful tool in basic and clinical systems neuroscience than before. A good example is the brain–machine interface using SMRs or evoked potentials such as P300, as will be discussed later. Furthermore, EEG (coupled with other methodologies) is an emerging method that takes advantage of the high temporal resolution of EEG and supplements spatial localization with other imaging methods. For example, simultaneous EEG and neuroimaging, especially fMRI, is an emerging method used to localize epileptic foci (Chaudhary et al. 2013).
5.3.1.2 Intracranial Recording
A number of invasive electrophysiological techniques have been established for clinical application. Electrocorticography (ECoG) is one such technique in which EEG can be recorded from an array of electrodes placed over the surface of the cerebral cortex. Typically, arrays of platinum electrodes are placed subdurally by means of surgery. ECoG has been the most reliable and powerful tool for localizing epileptic foci in patients with intractable epilepsy who are candidates for surgical removal of these foci. Another advantage of ECoG is that it can map functional areas of the cerebral cortex beneath electrode arrays such that surgical removal of the tissue does not damage functionally important (called “eloquent”) brain regions. This mapping can be carried out by electrical stimulation of the brain by means of electrodes or by recording evoked potentials in response to cognitive, motor, and sensory tasks. Brain mapping with ECoG provides complementary information when used with other brain-mapping tools such as fMRI (Hanakawa et al. 2001). Moreover, ECoG may clinically serve to bring about a high-performance brain–machine interface (BMI) (Yanagisawa et al. 2012) since the signal-to-noise ratio of ECoG is superior to that of scalp EEG.
Another clinically important type of intracranial electrophysiological recording can be obtained from electrodes implanted for deep-brain stimulation (DBS) to treat movement disorders. Recordings from DBS electrodes placed in the subthalamic nucleus or the globus pallidus have provided invaluable information about the pathophysiology of Parkinson’s disease. In particular, it has been shown that β-range oscillation is enhanced in the subthalamic nucleus and high-frequency stimulation suppresses the oscillation, thereby inducing clinical benefit in Parkinson’s disease (Kuhn et al. 2008).
5.3.1.3 Evoked Potentials and Event-related Potentials
Triggered averaging of EEG signals time-locked to repetitive sensory stimuli allows evoked potentials to be recorded by means of both scalp and intracranial recordings. Similarly, it is possible to record averaged EEG potentials time-locked to particular cognitive or motor events called “event-related potentials” (ERPs).
Sensory evoked potentials (SEPs) are evoked responses in the peripheral nerves, spinal cord, and brain following electrical stimulation of peripheral nerves such as the median nerve and the tibial nerve. SEPs are used to detect damage to the posterior column–medial lemniscus sensory system, which conveys touch, vibration, and proprioception. In patients with multiple sclerosis, sensory-evoked responses can be delayed or diminished. Conversely, patients with cortical myoclonus often show giant SEPs reflecting cortical hyperexcitability (Rothwell et al. 1984). Visual evoked potentials (VEPs) are responses to flash stimuli or visual pattern stimuli, and are recorded over occipital EEG electrodes. Abnormal VEPs may result from dysfunctions of the visual system including the visual nerves, optic tracts, lateral geniculate, optic radiation, and primary visual cortex. Short-latency auditory evoked potentials (AEPs) are often called “brainstem auditory evoked potentials” (BAEPs). BAEPs typically show seven positive peaks within 10 ms of click sounds. BAEPs are used to test brainstem function.
P300 is a positive ERP recorded approximately 300 ms after presentation of rare stimuli in an oddball paradigm in which frequent and rare stimuli are randomly presented and a participant is asked to count the number of rare stimuli. P300 can be applied to a type of BMI called the “P300 speller”, which detects brain activity related to the selection of stimuli such as letters (Farwell and Donchin 1988). Mismatch negativity is an ERP, also based on an oddball paradigm, which follows rare stimuli regardless of whether a participant pays attention to the stimuli or not. Mismatch negativity is reduced in patients with chronic schizophrenia (Nagai et al. 2013).
Movement-related cortical potentials (MRCPs) or Bereitschaftpotentials (BPs) are slow cortical potentials that precede voluntary movements. MRCPs/BPs are thought to originate from the supplementary motor area and primary motor cortex. Contingent negative variations (CNVs) are slow negative EEG potentials observed when a participant is anticipating an event of interest (Walter et al. 1964). CNVs are influenced by attention to an event.
5.3.1.4 Magnetoencephalography (MEG)
Much like EEG, the main source of MEG signals is synchronous postsynaptic currents in the pyramidal neurons of the cerebral cortex (Hari and Salmelin 2012). These electric currents accompany an electromagnetic field, which is theoretically detectable by coils outside the body. In contrast with the distorted electrical signals measured by scalp EEG as a consequence of poorly conducting skull bones, the magnetic field measured by MEG is not affected by the skull. MEG therefore shows great potential to localize the source of synaptic activity precisely. Although the electromagnetic field in the brain is very small (10–100 fT), the development of superconducting quantum interference devices (SQUIDs) has made detection of the electromagnetic field in the human brain possible (Cohen 1972). A helmet-type, whole scalp–covering MEG system with more than 300 SQUID sensors is currently available.
Note that MEG and EEG complement each other. The sensitivity of MEG to electromagnetic fields is different from that of EEG in that it depends on the direction of currents. MEG is most sensitive to currents tangential to the skull (i.e., postsynaptic activity occurring in the banks of cerebral gyri buried within the sulci/fissures), whereas EEG has sensitivity to signals from the depth of the brain and from the cerebral convexity. With these limitations in mind, electromagnetic source modeling that can provide information on the timing and direction of current flow is of great help in interpreting MEG data. The most straightforward approach has been to use an equivalent current dipole (ECD) model. A single ECD or multiple ECDs can be modeled. In minimum-norm estimates and minimum-current estimates (MNE/MCE), many small dipoles are placed throughout the cortex and their strengths are estimated as a function of time (Lin et al. 2006). In the beamforming procedure (Hillebrand et al. 2005), the volume of the brain is scanned by sequential application of spatial filters to pass activity from a specific brain area with maximum gain, while suppressing activity from other areas. Despite such progress, the currently available source analysis procedure cannot circumvent the inverse problem (i.e., the source of the electromagnetic field cannot be uniquely solved).
MEG has been applied to solving questions in basic systems neuroscience and cognitive/behavioral neuroscience including sensory perception, movement, language, social interaction, and development/learning. MEG can pinpoint the cortical generators of various evoked and event-related potentials that had been studied with EEG. MEG can also be applied to studying oscillatory brain activity and interareal connectivity. In the clinical domain, MEG plays an important role in epilepsy and preoperative mapping (Stufflebeam et al. 2009). MEG has also been applied to pathophysiological studies in movement disorders (Mima et al. 1998) and schizophrenia (e.g., mismatch field).
5.3.1.5 Assessment of Peripheral Nervous Systems and Muscles
Conventional electrophysiology techniques used to study functions of the peripheral nervous system and muscles are of importance, alone or in combination, to assess the peripheral functions of motor, sensory, and autonomic systems. It is also important to combine their use with modern neuroimaging and neurostimulation techniques.
Needle EMG is an important technique used to detect motor unit potentials. Active denervation can be diagnosed by detecting fibrillation potentials and positive sharp waves during muscle relaxation. Surface EMG is used to assess gross muscle activities. Integrated EMG has often been used to quantify gross muscle activities since it is proportional to isometric tension. Surface MEG is also used to record muscle activities evoked by electrical or magnetic stimulation of peripheral nerves and the motor cortex (nerve conduction studies, F-waves, H-reflex, etc.). It is possible to record surface EMG during neuroimaging (Hanakawa et al. 2002, 2003a), and during simultaneous brain stimulation and neuroimaging (Hanakawa et al. 2009; Shitara et al. 2011, 2013). Surface EMG helps assess the gross pattern of involuntary movement such as tremor, myoclonus, chorea, and dystonia. Therefore, neuroimaging studies on the pathophysiology of involuntary movement depend on surface EMG being monitored and recorded simultaneously and the data used as a covariate in the imaging analysis for proper interpretation of imaging findings.
5.3.2 Neuroimaging
Clinical systems neuroscience substantially relies on developing neuroimaging techniques that emerged in the final two decades of the twentieth century. Functional neuroimaging techniques include PET, single-photon emission computed tomography (SPECT), near-infrared spectroscopy (NIRS), and MRI. These techniques allow functional, neurochemical, and/or anatomical information to be obtained from healthy and diseased human brains non-invasively. These methods are occasionally called “brain-mapping techniques” since they are used to identify which parts of the brain represent a function of interest and then to create functional maps of the brain. The philosophy underlying such techniques is the assumption that a spatially segregated part of the brain code controls one or more distinct brain functions (functional segregation). Researchers in the field most often use Talairach and Tournoux’s (1988) coordinate system for the coordinates of brain maps. The system was originally (Talairach and Tournoux 1988) developed to assist neurosurgeons plan and execute stereotaxic surgery. A surface-based coordinate system has also been proposed to map the cerebral cortex (Dale et al. 1999; Fischl et al. 1999). However, functional segregation may not account for all the control mechanisms of cognition and behavior. It is important here to test the hypothesis that functions of the brain may primarily arise from interactions across different brain regions (functional integration). Note that functional segregation and functional integration are not mutually exclusive. Hence, it may be worth considering both types of control mechanisms whenever attempts are made to explain a particular ability of the brain before jumping to a conclusion.
5.3.2.1 Magnetic Resonance Imaging (MRI)
MRI is a medical imaging technique that makes use of nuclear magnetic resonance (NMR), in which nuclei in a magnetic field absorb and emit electromagnetic radiation. Nuclei with an intrinsic magnetic moment and angular momentum (such as 1H and 13C) can be tested with NMR, but currently available medical MRI systems almost exclusively use 1H because of the high prevalence of 1H protons in the body. Protons behave like microscopic magnets. Inside a strong static magnetic field, some protons are aligned parallel to the magnetic field and some are aligned anti-parallel to it. The number of parallel protons and anti-parallel protons are not equal; there are slightly more parallel protons than anti-parallel protons. This difference (approximately 2 × 1015 protons in a 1 × 1 × 5 mm3 voxel) produces a magnetization vector, which is a vectorial summation of all the magnetic protons in the voxel. ‘Voxel’ here refers to a unit in a regular grid in three-dimensional space, and is a unit of MRI acquisition. Now, protons have a resonance frequency of 128 MHz at 3 T (tesla). When a 128 MHz electromagnetic pulse is emitted from a transmit coil, protons absorb it and start to resonate in a 3 T MRI system. By creating a magnetic gradient and modulating electromagnetic pulses, MRI can tag the position of a voxel at which resonated protons emit electromagnetic radiation. Moreover, when the electromagnetic pulse disappears, the resonated protons start to emit electromagnetic radiation during a process that relaxes back toward the original condition. This is called the “relaxation process” during which electromagnetic radiation from protons can be detected by receiver coils.
There are a number of tissue factors influencing the relaxation process: longitudinal relaxation time (T1, spin–lattice) and transverse relaxation time (T2, spin–spin). T2 assumes a homogeneous magnetic field in the environment; however, a biological environment has magnetic inhomogeneity. For example, magnetic inhomogeneity may result from deoxy-hemoglobin (deoxy-Hb), which is a paramagnetic substance in red blood cells. The transverse relaxation time of tissue in the presence of magnetic inhomogeneity is called T2*, which is shorter than T2.
Conventional brain structural MRIs – such as T1-weighted, T2-weighted, proton density, and fluid-attenuated inversion recovery (FLAIR) images – have been of great importance in neuroradiological diagnosis of disorders. These images can detect atrophy, ischemic lesions, hemorrhages, space-occupying lesions, inflammation, metal deposition, and so forth. Clinical as well as scientific studies have made use of three-dimensional T1-weighted MRIs with fast spoiled gradient echo (FSPGR) or magnetization prepared rapid gradient echo (MPRAGE) sequences for volumetry, voxel-based morphometry (VBM), tensor-based morphometry (TBM), and cortical thickness measurement (Fig. 5.2). Gray matter volume or cortical thickness, as studied with these methods, has been shown to correlate with many aspects of human abilities (Hosoda et al. 2013). Recently, VBM has also been used as a method to elucidate neuroplasticity associated with development as well as use-dependent neuroplasticity associated with various kinds of learning and training in adults (Hosoda et al. 2013; Tanaka et al. 2013a). Gray matter/cortical thickness is also informative in clinical studies of dementia, movement disorders, and psychiatric disorders (Namiki et al. 2008; Yamada et al. 2007; Garraux et al. 2004, 2006).
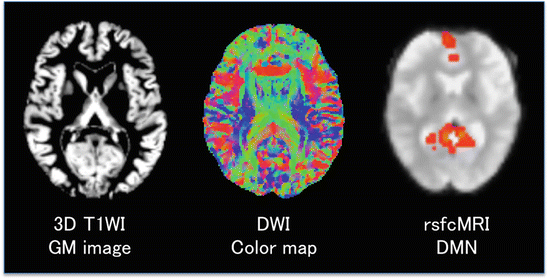
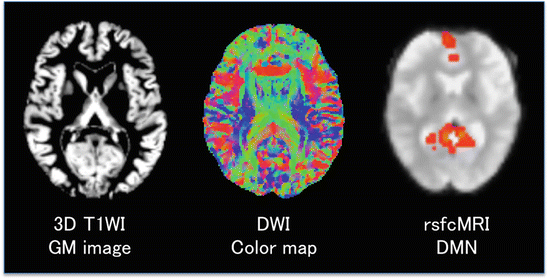
Fig. 5.2
Three representative images indicating different information obtained with MRI from a healthy volunteer. Left. A gray matter (GM) segmented image from a three-dimensional T1-weighted image. GM images have been used for VBM analysis of GM volume correlated with a cognitive function or differences in GM volume across groups. Middle. A diffusion-weighted image (DWI) measures the diffusion of water molecules. Assuming that the diffusion of water molecules reflects the orientation of fibers in white matter (WM), DWI can yield, for example, a color map simulating voxel-wise predominance of WM fiber orientations (red = right–left fiber orientation; green = anterior–posterior; blue = superior–inferior). Right. Resting-state functional connectivity MRI (rsfcMRI) shows the medial prefrontal area and posterior cingulate area (red) corresponding to parts of the default mode network (DMN). An independent component analysis (ICA) was used to separate different resting-state networks (RSNs) in an rsfcMRI dataset without a priori hypothesis. The DMN (red) is overlain onto a gradient-echo echo planar image used for acquisition of rsfcMRI data
Emerging MRI methodologies comprise a number of diffusion-weighted MRI (DWI) techniques (Fig. 5.2, Middle) such as diffusion tensor imaging (DTI), diffusion kurtosis imaging (DKI), diffusion spectral imaging (DSI), and q-space imaging (QSI). DWI can be used to visualize the diffusion of protons, especially those of water molecules. It has had a major impact on clinical practice in detecting early stroke (Moseley et al. 1990). DTI-derived images – such as fractional anisotropy (FA) and mean diffusivity (MD)/apparent diffusion coefficient (ADC) – allow quantitative analysis of changes in water diffusion associated with morphological change and damage to brain tissue. Moreover, diffusion-based fiber tractography has proven to be useful in assessing macroanatomical fiber connections in both healthy and diseased people. For example, DWI-based tractography is used to determine the dominant hemisphere (Matsumoto et al. 2008) and guide neurosurgical procedures (Okada et al. 2006). It is also possible to assess longitudinal changes in DWI-derived parameters resulting from neuroplastic changes associated with learning (Hosoda et al. 2013).
Blood oxygenation level–dependent (BOLD) fMRI is a functional neuroimaging method that uses deoxy-Hb as an internal contrast agent (Ogawa et al. 1992). To date, it has mostly been carried out using T2*-weighted MRI such as gradient-echo echo planar imaging (GE-EPI). BOLD fMRI uses T2* signals as a surrogate measure of synaptic/neuronal activations (see the “Neurometabolic–Hemodynamic Coupling” section later in the chapter). For more than two decades, BOLD fMRI has been the most widely and intensively used neuroimaging/brain-mapping tool, despite the cost of establishing and maintaining an MRI facility. There are several reasons for this. First, BOLD fMRI has the finest spatial resolution (typically, a few millimeters) of all the currently available non-invasive brain-mapping tools. Moreover, it provides the most accurate localization in the whole brain from the cortical surface to the deep nuclei and cerebellum. Nowadays, spinal cord fMRI is also feasible. fMRI has a time resolution (typically, a few seconds) which is sufficient to capture BOLD signal time courses after a brief stimulus. Second, structual MRI taken together with fMRI can obtain a variety of structural information with high spatial resolution as described above (such as three-dimensional T1-weighted images). Third, BOLD fMRI is capable of studying not only functional segregation but also functional integration (functional connectivity and effective connectivity) by using either psychophysiological interactions (Friston et al. 1997; Abe et al. 2007; Aso et al. 2010) or dynamic causal modeling (Friston et al. 2003). Task-related fMRI has been applied to an enormous number of issues in systems/cognitive/behavioral neuroscience: sensory perception (Ban et al. 2013; Yamamoto et al. 2008; Aso et al. 2007; Sawamoto et al. 2000), movement (Hanakawa et al. 2003a, 2006, 2008a; Toma et al. 1999), language (Nakamura et al. 2000a, 2002; Crinion et al. 2006; Hosoda et al. 2011), imagery (Hanakawa et al. 2003a, 2008b), attention (Nakamura et al. 2000b), cognition (Hanakawa et al. 2002, 2003b, c; Abe et al. 2007; Kasahara et al. 2013), decision making (Fukui et al. 2005), and social interaction (Shinozaki et al. 2007; Fukui et al. 2006). Similarly, task fMRI has been applied to a variety of disorders involving the central nervous system (Tanaka et al. 2012; Oga et al. 2002; Bohlhalter et al. 2006). Note, however, that interpretation of task fMRI results is often difficult because task difficulty and task performance are often significantly different between a healthy group and a patient group. To circumvent this problem, recent attention has been focused to resting-state functional connectivity MRI (rsfcMRI) in which participants undergo no particular task (Fox and Raichle 2007; Smith et al. 2013). This variation of fMRI depends on ultra-slow fluctuations (0.01–0.1 Hz) of BOLD signals, which perhaps reflect changes in synaptic/neural activities occurring spontaneously across the nodes of a functional network. Thus, without any tasks, rsfcMRI can identify motor, visual, attention, auditory, and default mode network (DMN) systems (Raichle et al. 2001) (Fig. 5.2, Right). For example, abnormalities in rsfcMRI have been identified in dementia, schizophrenia, mood disorders, epilepsy, and movement disorders (e.g., Greicius 2008).
As a result of fMRI having a powerful magnetic field, it has previously been difficult to acquire fMRI data simultaneously with other recording techniques. However, technical advances have made it possible to combine fMRI with many other techniques such as transcranial magnetic stimulation (TMS) (Hanakawa et al. 2009; Shitara et al. 2011, 2013) and EEG (Omata et al. 2013). In particular, simultaneous EEG–fMRI has the potential to become an important tool in clinical research of epilepsy (Chaudhary et al. 2013).
5.3.2.2 Positron Emission Tomography (PET)
PET is a nuclear medicine imaging technique that uses a positron-emitting radionuclide-labeled tracer. It can create a three-dimensional functional image of the body including the brain. The labeled tracer is typically injected into the bloodstream, where the tracer is distributed throughout the body according to its biochemical characteristics. The positron-emitting radionuclide emits a positron, which travels a short distance (~1 mm) in tissue and interacts with an electron. When the two particles meet, they annihilate each other and emit annihilation radiation (a pair of 511 keV gamma photons emitted in opposite directions). This pair of gamma photons simultaneously reaches scintillators on the scanner and produces a burst of light, which is detected by photomultiplier tubes. Detection of this coincidence event allows PET to accurately localize the distribution of the tracer in the brain. After collecting many coincidence events, a tomographic reconstruction method is applied to yield a three-dimensional image dataset.
A shortcoming of PET is radiation exposure. Another is the expensive cost of a PET facility, which is typically equipped with a PET scanner(s), a cyclotron, and a laboratory for tracer synthesis. This is because many positron-emitting radionuclides used for brain PET have short lives, and therefore tracers must be synthesized just before use. Nevertheless, PET is a powerful and indispensible functional imaging tool for both the basic and clinical domains of systems neuroscience. One of the strengths PET has over other imaging modalities is its high signal-to-noise ratio. Another is the capability of PET to obtain specific molecular information depending on the tracer in use (so-called “molecular imaging”). Brain PET images using 18F-labeled fluorodeoxyglucose (18F-FDG) reflect glucose metabolism levels associated with regional neural/synaptic activities. Reduced levels of 18F-FDG indicate pathological changes in brain metabolism associated with neuropsychiatric disorders such as epilepsy (Takaya et al. 2006) and Alzheimer’s disease (Weiner et al. 2010). Moreover, the development of tracers binding to β-amyloid, such as Pittsburgh compound B (PIB), has dramatically changed PET diagnosis of Alzheimer’s disease, preclinical Alzheimer’s disease, and mild cognitive impairment (MCI) (Weiner et al. 2010). The development of new amyloid tracers is currently ongoing. Other tracers detecting neuroaggregates (e.g., tau) in the human brain are also close to being approved for clinical application.
PET also allows neurotransmitter imaging of many types: dopamine receptors (11C-raclopride and 18F-fallypride), enzymes related to dopamine synthesis (18F-dopa), acetylcholine synthesis (11C-methylpiperidin propionate), and serotonin transporters (11C-DASB).
15O-labeled water is distributed in the brain in proportion to regional cerebral blood flow (rCBF). Changes in rCBF are connected with changes in neural/synaptic activities (as will be discussed later in Sect. 5.3.3). Thus, brain PET images of H2 15O distribution during a particular task can identify brain regions neurally activated during the task in healthy (Hanakawa et al. 2002; Lerner et al. 2009) and diseased people (Lerner et al. 2004, 2007; Sawamoto et al. 2007). Such PET activation studies are also possible to test dopamine release during a particular task. Internally released dopamine competes with externally administered 11C-raclopride for dopamine receptor D2 binding. Therefore, reduced 11C-raclopride uptake during an experimental task compared with a control task indicates increased dopamine release in the experimental task (Sawamoto et al. 2008).
Silicon avalanche photodiodes have replaced conventional photomultiplier tubes in recent PET systems. Since they function within a strong magnet, it is now possible to combine PET and MRI as a hybrid PET/MRI system using a single scanner device.
5.3.2.3 Single-photon Emission Tomography (SPECT)
SPECT is another nuclear medicine imaging technique that uses a gamma-emitting radioisotope. A SPECT machine is usually equipped with multiple gamma cameras to acquire multiple two-dimensional images from multiple directions. After image acquisition, a tomographic reconstruction method is applied to yield a three-dimensional dataset. Alhough SPECT has lower spatial resolution than PET, it is less expensive.
SPECT has been used to measure brain perfusion using 99mTc-HMPAO (hexamethylpropylene amine oxime) or 99mTc-ECD (ethyl cysteinate dimer). Perfusion SPECT can detect changes in diffuse perfusion abnormality in dementia. It can also detect focal changes in cerebral vascular disorders. Perfusion SPECT has a particular application in functional neuroimaging. PAO and ECD are fixed within the brain a few minutes post injection using the so-called “snapshot method.” Therefore, SPECT provides a unique opportunity to study mechanisms underlying walking difficulty in neurological disorders. Patients with Parkinson’s disease show underactivation of the supplementary motor areas as they walk (Hanakawa et al. 1999a), whereas they show overactivity in the lateral premotor cortex during paradoxical improvement of walking under appropriate visual guidance (Hanakawa et al. 1999b). A follow-up study into vascular parkinsonism replicated dysfunctions in supplementary motor areas and compensatory activity in the lateral motor cortex during parkinsonian walking (Iseki et al. 2010).
SPECT with 123I-ioflupane (FP-CIT SPECT) can now be used for dopamine transporter (DAT) imaging to visualize nigrostriatal dopaminergic nerve terminals. Parkinson’s disease and dementia with Lewy bodies show reduced DAT signals in the striatum (putamen and caudate nucleus).
5.3.2.4 Near-infrared Spectroscopy (NIRS)
NIRS uses the near-infrared region of the electromagnetic spectrum (700–1400 nm) and measures absorbance at specific wavelengths corresponding to oxyhemoglobin (oxy-Hb) and deoxy-Hb. NIRS devices come with a pair of emitters and a probe detector attached to the scalp. Near-infrared light in the 700–900 nm range of the spectrum penetrates the scalp and skull, and then reaches the surface of the brain. In this range of the spectrum, oxy-Hb and deoxy-Hb are strong absorbers of light. Therefore, NIRS can measure the relative concentration of oxy-Hb and deoxy-Hb from the brain surface. The detector typically measures reflected (backscattered) light in which photons may take a variety of paths, often modeled as an ellipsoid in shape. The distance between the emitters and the detector (typically 2–3 cm) determines the size of the ellipsoid. The modified Beer– Lambert law is used to calculate micromolar-level changes in oxy-Hb and deoxy-Hb tissue.
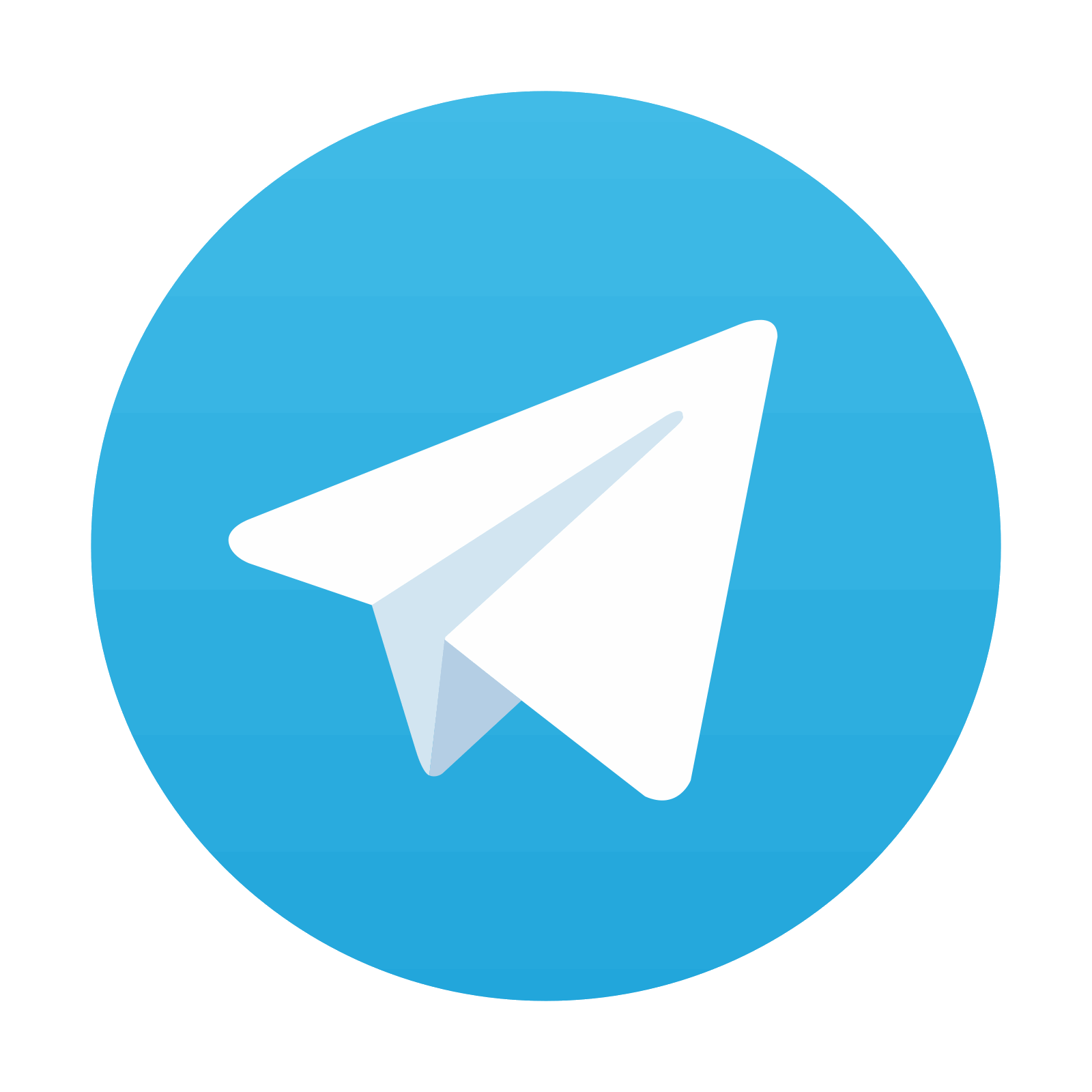
Stay updated, free articles. Join our Telegram channel
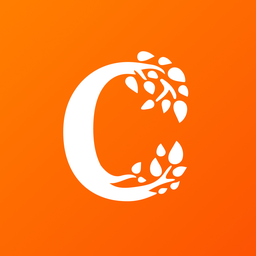
Full access? Get Clinical Tree
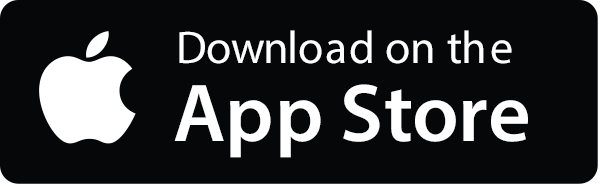
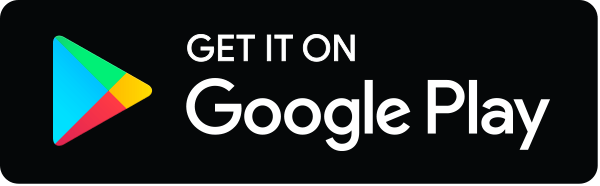