Chapter 6
Congenital Deformities
6.1Etiology of Congenital Spine Anomalies
6.2The Clinical Spectrum of Deformity
6.8Congenital Spinal Deformity Syndromes
6 Congenital Deformities
6.1 Etiology of Congenital Spine Anomalies
It is well established that abnormalities of mesodermal development may occur in association with congenital anomalies of the spinal cord and meninges. Various theories have been proposed for the embryological derivation of such deformities of the neurospinal axis. Conditions such as spina bifida and diastematomyelia and anatomical aberrations such as butterfly vertebrae, hemivertebrae, failures of vertebral segmentation, and paravertebral and postvertebral cysts have all been explained in terms of erroneous differentiation of the early embryonic mesodermal and ectodermal cell layers. It is thus not difficult to see how a spinal deformity can arise from such identifiable embryopathic abnormalities.
Associations of deformities of the gut in patients exhibiting congenital defects in either one or both components of the neurospinal axis have alerted investigators to the existence of an intricate relationship between the systems. 1– 5 As a result, it is now a commonly held opinion that all types of clinical syndromes with anomalies in both spinal and intestinal structures originate from one basic complex of anomalies and finally become diversified by the modifying effects of growth and repair.
6.1.1 Normal Development of Axial Structures
The early embryo consists of two epithelial cell layers: ectoderm (epiblast) and endoderm (hypoblast). In experiments involving separation, rotation, and recombination of these two layers in duck embryos it has been demonstrated that it is the endoderm that controls the position of the primitive streak in the overlying epiblast and is thus instrumental in establishing the primary (anteroposterior) axis. 6 Mesoderm (primary mesenchyme) cells formed in the primitive streak migrate laterally and anteriorly between the two epithelia, while the notochord extends forward from the anterior end of the streak, at first forming as a flattened structure closely applied to the endoderm, which is the future dorsal wall of the embryonic gut tube. The streak itself remains at the posterior end as the embryo elongates (▶ Fig. 6.1).
Fig. 6.1 Stages in the normal development of axial structures. (a) Cells migrate between the two layers of the embryonic bilaminar disk to form mesoderm. (b) The notochord flattens forming the notochordal plate which comes to lie in intimate contact with the roof of the endoderm; at this stage a transient connection, the neurenteric canal, exists across the embryonic disk. (c) The notochord reforms and the axial mesenchyme segments to form paired somites.
The mesoderm cells adjacent to the notochord (paraxial mesoderm) form a series of paired segmental epithelioid blocks, the somites, except in the major part of the cranial region. These cells establish the segmentation of the embryonic body, on which the segmental pattern of the skeletal, muscular, nervous, and vascular systems of the adult trunk is based. The regularity of their pattern and their relationship to the notochord and developing neural tube are therefore of fundamental importance.
The ectoderm overlying the paraxial mesoderm differentiates into the neural plate, which changes shape to form the neural groove then neural tube, coming to lie internally. The whole surface of the embryo is thus formed from ectoderm, which is initially lateral to the neural plate. Following the closure of the neural tube, the epithelial structure of the medial surface of the somites breaks down and the cells are reorganized as mesenchyme. They migrate to surround the notochord, which has by this time separated from the gut wall to form a rod-shaped structure. The segmental pattern of this sclerotomal mesenchyme is retained, but the primordia of the vertebrae thus initiated form in a position that is staggered relative to the part of the somite left behind to form segmental muscle blocks. Thus vertebrae are described as intersegmental in position, whereas the spinal nerves and myotome-derived muscles are segmental. 7, 8 The regularity and bilateral symmetry of this arrangement are essential to normal axial development (▶ Fig. 6.2). 9
Fig. 6.2 Condensation of the mesodermal somites occurs such that definitive vertebral bodies derive from parts of the adjacent somites.
According to observations originally based on light microscopy of paraffin sections, the human embryonic notochord differs from its rodent counterpart in being, in its early stages, a hollow structure. The neurenteric canal forms a communication between the amniotic cavity dorsal to the ectoderm and the yolk sac (prior to formation of the gut tube) ventral to the endoderm. This communication from the gut to the dorsal surface of the embryo passing through the midline dorsal structures is a normal but very short-lasting connection and its final location in the fully developed human is at the tip of the coccyx. 2
6.1.2 Theories of the Developmental Basis of Deformities
Dodds 10 suggests that an abnormally large neurenteric canal, or one that persists too long, would prevent fusion of the converging notochordal and sclerotomal streams. This anomaly could therefore be responsible for the anterior vertebral clefts that most commonly involve the cervicodorsal spine, and for neurenteric connections, cysts, and diverticula, as well as reduplications of the spinal cord. The final location of this neurenteric canal, however, is at the tip of the coccyx, and Bremer 2 suggests that any connection between the intestinal tract and midline dorsal structures above the coccyx is due to the previous existence of an accessory neurenteric canal. He further points out that the former presence of such an accessory canal may be recognized as one of the neurenteric connections mentioned above.
An alternative explanation of this embryonic system interrelationship is presented by Fallon et al, 3 who suggest the notochord, at an early stage of development, becomes intercalated with the endoderm. It is postulated that if the notochord becomes incompletely separated from the endodermal lining then abnormalities of the vertebrae might well occur, since the mesoderm would be unable to surround the notochord completely.
Saunders 11 proposes that a local cleavage of the notochord, for whatever reason, enables endoderm and ectoderm to adhere and form a neurenteric band and/or enteric diverticulum. Such a structure may or may not elongate with growth and so interfere with development of either system by causing traction diverticula, cysts, or strands which may or may not be expressed as vertebral defects. It is an interesting feature of thoracic diverticula and cysts connected with the stomach or intestines that they have a tendency to lie to the right of the vertebral column 3; this is thought to be caused by a dextrolateral displacement of the embryo’s neurenteric connection by the developmental processes of gastric and gut rotation. Bentley and Smith 5 develop this theory further and classify a wide variety of malformations under the general concept of split notochord syndrome. They emphasize that differential growth of gut and vertebral column could lead to wide separation of the areas affected in each.
Beardmore and Wigglesworth 4 propose as a primary event a local endodermal–ectodermal adhesion to occur in the midline of an embryo at a presomite stage between Hensen’s node and the prochordal plate. They postulate the adhesion to partially block the cephalad invagination of notochordal material, causing a splitting or diverting to either side of the notochord. In this way various developmental defects of the vertebrae could arise. Prop et al 12 extend this view to the second phase of notochordal growth and suggest that the adhesion might be located in the primitive streak—that is, more caudally.
Although there are some differences in the primary events, the explanations described above have in common the concept of abnormal relationships between notochord and endoderm (including notochordal cleft), and ectodermal–endodermal connections in some stages (▶ Fig. 6.3).
Fig. 6.3 Embryonic adhesions between endoderm and ectoderm at an early stage can give rise to a number of combined anomalies of gut and spine.
Abnormalities occurring during this crucial, initial period of spinal development can have catastrophic consequences. They are often fatal, although at least they can result in the development of major vertebral deformities with significant neurological impairment. The etiological factors responsible for such abnormalities remain obscure and no single genetic or environmental defect has been identified. Recent work by Smithells et al, 13 however, has highlighted the importance of maternal nutrition with particular regard to vitamin intake in the periconceptual period.
6.1.3 The Pathogenesis of Congenital Vertebral Malformations
Congenital malformations of vertebrae can be divided into failure of formation, failure of segmentation (▶ Fig. 6.4), and the spectrum of abnormalities associated with developmental anomalies of the notochord. Both failure of formation and failure of segmentation can take the form of defects or errors. Failure of formation refers to abnormalities of the vertebral bodies and can be subdivided into (a) a partial or total defect (absence) of the body, and (b) abnormalities in shape. Failure of segmentation refers to abnormalities of the intervertebral disk and can also be subdivided into (a) simple defect (absence) of the disk either totally as in block vertebrae or partially as in a unilateral bar, and (b) errors in disk position or alignment. Many explanations as to how these developmental abnormalities are produced have been proposed, although there is as yet no general agreement as to their origins.
Fig. 6.4 Diagram to show the classification of congenital spinal. anomalies. (Reproduced with permission from Pediatric Spine, 2nd Edition, Ed S L Weinstein, Lippincott WW, PA 2001; Chapter 7, Table 1, p. 163).
Failure of Formation
Feller and Sternberg 14 postulated that failure of formation (hemivertebra) was due to the lack of a cartilaginous precursor secondary to any underlying problem with the notochord (▶ Fig. 6.5). Junghanns 15 proposed that a lack of ossification resulting from inadequate vascularization was responsible, although his interpretation was based on the concept that the vertebral body in the early stages of chondrification consisted of two halves separated by a ventrodorsal extension of the perichordal sheath and that ossification centers appeared both dorsally and ventrally. Thus lateral hemivertebrae arose from failure of ossification on one side following failure of fusion of the two chondrification centers. Ventral or dorsal hemivertebrae were thought to arise due to lack of ossification secondary to lack of vascularization.
Fig. 6.5 PA radiograph showing a hemivertebra: a unilateral failure of formation.
Although Bardeen 16 described two chondrification centers divided by the perichordal septum, no confirmatory descriptions are given in later papers. 7, 17, 18 The more recent detailed studies of Tanaka and Uhthoff 9, 19 failed to find any evidence to support the mechanism put forward by Junghanns. The vertebral body was found to form as a single cartilaginous structure and defects that were not limited to exactly half or the whole of the body were already present at the stage of chondrification and could not therefore have resulted from failure of ossification. Although failure of formation could arise from abnormal migration of sclerotomic cells, these do not show any morphological differences other than in density. It is now thought that formation defects arise as a result of abnormal differential growth of mesenchymal cells in the stage of resegmentation.
Defects of the vertebral body or anomalies in its shape can result in compensatory growth of adjacent vertebrae. Since ossification centers develop in a normal oval form even in malformed vertebral bodies, the shape cannot be fully determined radiologically until ossification is complete; however, abnormalities in shape can be recognized at much earlier stages. 19
Failure of Segmentation
The mechanism of failure of segmentation is also controversial. Junghanns did not comment on it; Valentin and Putscher 20 considered that it was due to destruction of the intervertebral disk after it had been formed; Ehrenhaft 21 believed that complete chondrification of the mesenchymal column was responsible; Overgaard 22 thought it arose from regressive changes at the end of the developmental period; and Tsou 23 suggested that it resulted from osseous metaplasia of the annulus fibrosus. Tanaka and Uhthoff 9 describe one 70-mm fetus where failure of segmentation resulted from failure of development of the intervertebral disk before or at the early stages of chondrification. Normally the mesenchymal cells in the dense-celled area start to differentiate into the fibroblastic cells of the annulus shortly after chondrification of the vertebral body has been completed; thus failure of segmentation is due to either complete chondrification of the dense-celled area or its absence altogether (▶ Fig. 6.6).
Fig. 6.6 PA radiograph showing a unilateral bar: a unilateral failure of segmentation.
Junghanns stressed the importance of vascularization in the etiology of developmental abnormalities of vertebrae. 15 Although more recent studies have indicated that defects arise at much earlier stages of development than suggested by him, they have confirmed the crucial role of a normal blood supply. It would appear that abnormalities of formation and segmentation occur during the stage of resegmentation of the mesenchymal vertebral column and are related to abnormalities of the intersegmental arteries. There is no doubt about the relationship between congenital anomalies and a reduction or even absence of local blood supply.
Malformations Associated with Anomalies of the Notochord
The influence of the notochord on vertebral development has been in dispute. Feller and Sternberg 14 described a curvature of the notochord toward hemivertebrae and believed that the notochord was responsible for malformations of the vertebral body.
Schmorl and Junghanns 24 felt that persistent notochordal tissue hinders vertebral development. Tanaka and Uhthoff 19 report one specimen with bifurcation of the notochord in the absence of any malformation, although the intersegmental arteries were normally distributed, suggesting that no relationship exists between malformations of the vertebral body and the notochord.
There is, however, an undoubted collection of related developmental abnormalities of the skin of the back, the central nervous system, the spine, and the gut for which there is much evidence, albeit mainly from studies of the embryos of lower vertebrates, that the etiology lies in abnormal development of the notochord. These abnormalities have been collectively referred to by Bentley and Smith 5 as the split notochord syndrome (▶ Fig. 6.7).
Fig. 6.7 The range of abnormalities attributable to the split notochord syndrome.
Lereboullet 25 described embryos split sagittally with the yolk sac being exposed in the dorsal cleft following artificial insemination of pike ova. Hertwig 26 produced ring embryos with dorsal clefts as a variant of the neurenteric canal by fertilizing postmature frog ova. He appreciated the role of these abnormal communications in the production of combined spina bifida and coined the word diastematomyelia. Clinical cases of combined spina bifida had already been described, 27– 29 and Gruber reported cases of spinal defects associated with intestinal fistulae. 29 Johnston 30 and Frazer 31 both reported notochord clefts in human embryos, and Warkanay 5 has experimentally produced changes in mammalian embryos similar to those seen in the frog ring embryos of Hertwig. 26
According to Bentley and Smith, 5 partial duplication and separation of the notochord leads to herniation of the yolk sac and its adherence to the dorsal ectoderm. The hernia may rupture to produce a fistula that separates the future spine and cord into two halves. Subsequent growth attempts to close and obliterate the fistula meet with a variable degree of success upon which the final deformity depends. Development of the base of skull, vertebral column, and central nervous system as well as the gut may be affected. Sequestration of dorsal ectoderm can produce dorsal dermoid cysts and sinuses, and remnants of the yolk sac may differentiate into tissue characteristic of any part of the gut or its embryological derivatives and persist as posterior enteric remnants in the form of fistulae, sinuses, diverticula, or cysts. Differential growth of the vertebral column and gut leads to wide separation of the affected regions and abnormal fixation of bowel may lead to failure of rotation.
When closure of the defect in the spinal column is incomplete the resultant spinal anomaly can range from a slight widening of the vertebral body to a complete anterior and posterior spina bifida. Minor degrees of anterior spina bifida can produce the radiological appearance of butterfly vertebrae (▶ Fig. 6.8); more severe lesions may incorporate enteric or cutaneous tissues. Hemivertebrae can undergo distorted individual growth, their medial pedicles fusing to form the bony spur characteristic of diastematomyelia. In all these malformations the spinal canal is wider than normal and the meninges may protrude anteriorly or posteriorly where the spine is bifid. When the spinal cord is itself bifid (diplomyelia) then the two halves are separately invested with meninges. The more usual arrangement is for the cord to be divided into right and left halves (diastematomyelia) with no nerve roots arising medially from either half. The more common variety of posterior spina bifida is the result of a separate abnormality occurring much later on in development, although in both types there may be hamartomatous changes in the overlying tissues, usually in the form of a lipoma, an angioma, an area of hypertrichosis or hyperpigmentation, or a dermal pit or sinus (▶ Fig. 6.9). Recently it has been noted that haploinsufficiency of Notch signaling pathway genes in humans can cause these problems and along with short-term gestational hypoxia significantly increases the penetrance and severity of vertebral defects in a mouse model. 32
Fig. 6.8 A typical butterfly vertebra.
Fig. 6.9 A midline patch of hair is a common accompaniment to an underlying congenital spinal anomaly.
6.2 The Clinical Spectrum of Deformity
The term congenital spine deformity implies one that is present at or before birth. This is not strictly true. While the underlying anomaly is most certainly congenital, it does not necessarily produce a spinal deformity at all, and some of those that are produced only manifest themselves years later. The classification of congenital spine deformity is therefore semantically incorrect, as what is being classified is the underlying vertebral anomaly. There are two basic types of congenital spine anomaly capable of producing a spinal deformity: congenital bone anomalies and congenital cord anomalies (myelodysplasia).
6.3 Congenital Bone Anomalies
Two types of congenital bone anomaly commonly produce a deformity: failure of formation and failure of segmentation. The direction of the subsequent deformity is initially entirely dependent on which part of the spine is involved (the side, front, or back). Thus lateral defects produce a scoliosis, anterior defects a kyphosis, and posterior defects a lordosis. Such purely unidirectional deformities are uncommon, as the anomaly and asymmetric growth usually occur in more than one plane. Therefore, a three-dimensional approach to the understanding of the behavior of congenital spine deformities is essential. In addition, thorough knowledge of the pathomechanics of idiopathic scoliosis is necessary so that changes in direction with growth can be understood with congenital and some other etiologies (see ▶ 3). 33– 36
Lordotic deformities are much more common than appreciated, particularly with failures of segmentation and thus with reduction of the growth of the back of the spine; on the concave side they provide the rotational potential in the same mechanical fashion as in idiopathic scoliosis. Rotation with the posterior elements always directed toward the curve concavity testifies to the presence of the lordosis. Moreover, progression from birth creates very serious problems, as chest rotation with diminution of the anteroposterior chest diameter may cause severe cardiopulmonary compromise in exactly the same way as idiopathic scoliosis of early onset. Interestingly, if such areas of congenital lordosis exist in an area that resists the tendency to rotate, such as the cervicothoracic junction or upper thoracic spine, then the rotational effect is translated down into the thoracic spine to produce a secondary deformity. It is therefore not uncommon to encounter an idiopathic-type curve below a seemingly innocuous congenital anomaly higher up. Indeed, this is precisely what has happened, the congenital anomaly producing the necessary essential lesion of biplanar asymmetry 36 (▶ Fig. 6.10).
Fig. 6.10 (a) PA radiograph showing a hemivertebra at T8/9 and failures of segmentation above that. (b) Within 3 years these thoracic congenital abnormalities have thrown off a large idiopathic-type curve below.
If the median sagittal plane asymmetry produced is a kyphosis then the spine is protected from rotation. Any associated coronal plane asymmetry can only progress with asymmetric vertebral growth in this plane with very much less of a scoliotic deformity than occurs with idiopathic scoliosis. The spinous processes will, however, be neutral or directed toward the curve convexity, as must occur with an asymmetric kyphosis.
6.3.1 Congenital Scoliosis
Natural History
Just as progression potential is crucial in idiopathic scoliosis so it is in congenital deformities but with the latter there are several different anomaly types to be considered. In general, it can be said that about a quarter of cases are nonprogressive, a quarter are mildly progressive (not more than 30 degrees), and half are significantly progressive (more than 30 degrees). There have been many studies on untreated cases of congenital spinal bony anomalies but, quite naturally, the bigger series have tended to come from the bigger centers, such as, 234 cases from Minneapolis, 37 315 from Greenville, 38 and 251 39 from Edinburgh. With the two basic types of congenital anomaly—failure of formation or failure of segmentation (▶ Fig. 6.4)—in the former, part or the whole of one side of the vertebra does not develop leaving behind a wedge vertebra or a hemivertebra, respectively. 40 With the latter, a failure of segmentation, whereby the intervertebral disk and of course the growth plates on each side fail to develop either all the way across, or part way giving rise to respectively a bilateral failure of segmentation (block vertebra) or unilateral failure of segmentation (unilateral bar), the latter usually a significantly progressive problem. 37, 39 How these are then distributed within the same spine provides detail and prognosis of the problem.
Thus, scoliosis progression potential would appear to depend on the type, site, and extent of the anomaly in the spine and its effect on vertebral growth (worse during the infantile and preadolescent/adolescent growth spurts). If there are multiple congenital anomalies and you are uncertain what the future holds then it is quite reasonable to merely follow the patient up. This 1-year-old had a scoliosis of 33 degrees with a bar on the right at the T4/5 level and a probable bar on the left at the L1–3 level with other obvious anomalies (▶ Fig. 6.11).
Fig. 6.11 (a) PA X-ray of an infant of 1 year old. The natural history was uncertain and so he was regularly reviewed. (b) At the age of 16 he had the same scoliosis of 33 degrees! Masterly inactivity is amazingly therapeutic.
Since Kleinberg first described the bad prognosis of the unilateral failure of segmentation, 41 multiple unilateral anomalies have become recognized as the most progressive type. 42 This applies to multiple unilateral failures of either segmentation or formation. 43, 44 A particularly sinister combination is the unilateral bar on one side and a hemivertebra on the opposite, 38 the one compounding the other (▶ Fig. 6.4). By contrast balanced anomalies protect against progression, 44 such as, a hemivertebra on one side and a nearby hemivertebra on the other side (hemimetameric shift). Although it is said that solitary hemivertebrae do not tend to produce significant deformities unless they are free (nonincarcerated) 37, 39, 40 or occur in the lumbar or lumbosacral regions throwing the trunk out of balance this is often not so and they can produce deformities difficult to deal with unless treated early (▶ Fig. 6.10 and ▶ Fig. 6.12).
Fig. 6.12 A typical lumbosacral hemivertebra which immediately throws off a left-sided curve above.
The most common type of congenital scoliosis encountered is due to a unilateral failure of segmentation followed by a hemivertebra, a bar and contralateral hemivertebra, a block vertebra, and, least common, a wedge vertebra. 39 The anomalies in descending order of progression potential are, however, bar and contralateral hemivertebra, bar only, hemivertebra, wedge vertebra, and block vertebra. 39 Girls are more often affected than boys and appear to have a worse progression potential. Progression is more obvious if the anomaly exists low in the thoracic region or in the thoracolumbar region, and least obvious high in the thoracic region. 37, 39, 40 Once a deformity starts progressing it never stops during growth. Deformities presenting shortly after birth have a worse prognosis and progressive deformities of all types tend to deteriorate more during the preadolescent/adolescent growth spurt. However, about a third of cases have multiple anomalies and are difficult to classify 37, 39, 40 (▶ Fig. 6.13).
Fig. 6.13 Multiple congenital anomalies nicely demonstrated by 3D CT scanning. It looks as though there is a hemivertebra at T9 and above that two block vertebrae. Meanwhile the apex on the concave side shows clearly a rib fusion which means there is a failure of segmentation opposite the hemivertebrae.
Asymmetric growth in the coronal plane has been regarded as the most important factor governing progression potential. 37, 39, 40 Indeed it would appear obvious that no growth is possible on the side of the bar while some growth is still available on the concave side of a hemivertebra, but is that the sole reason why the former has the worse prognosis? Moreover, it is well known that not all radiographically similar deformities behave in the same way. 44 In order to fully understand progression potential it is necessary, as it was with idiopathic scoliosis, to encompass the median sagittal plane and consider the spine in all three dimensions. While the anomalies producing congenital scoliosis have been exhaustively classified and monitored in the coronal plane, it is the biomechanical effect of the sagittal plane which holds the key.
The Importance of the Sagittal Plane
A clue to the importance of this plane is derived from the observation that deformities associated with failures of segmentation have a severe progression potential. When an area of the spine fails to segment it can do so both at the front and at the back and, indeed, evidence of a posterior plaque is commonly seen radiographically (▶ Fig. 6.14), particularly in the older child when ossification is more advanced. If this segmentation failure is unilateral then the more obvious coronal plane anomaly is compounded by a posterior tether, thus the same biplanar mechanism exists as in idiopathic scoliosis. 36 This is verified by the fact that the spine rotates in a constant direction with the posterior elements directed toward the curve concavity (concordant rotation), which can only occur with a rotated lordosis. The more levels that are affected then the more both components of the deformity are augmented and the more serious is the rotational progression. The reason why not all patients with seemingly the same radiographic anomaly have the same rate of progression is not because of differential growth in the frontal plane 44 but because the more important posterior tether is clearly variable with the degree of rotational instability more or less pronounced.
Fig. 6.14 PA radiograph showing that, from T11 to L1 on the left side, a posterior plaque is seen. Note there is no rib fusion that would occur with a vertebral body failure of segmentation, because ribs are derived from the costal processes of the vertebrae.
Conversely, failures of formation tend not to have the same “tethering” effect posteriorly and, thus, while the deformity may progress in the coronal plane, it is less often associated with rotation and so progression potential tends to be less marked. However, when a failure of segmentation is sited opposite to a failure of formation (▶ Fig. 6.13)—a notoriously progressive situation 37, 39, 41—then the biplanar asymmetry so produced facilitates a marked degree of rotational progression.
It has also been stated that a bilateral failure of segmentation should theoretically not produce a scoliosis 37 and yet sometimes, apparently surprisingly, a curvature is seen. This is due entirely to the sagittal plane. Two, or more, vertebrae that are fused together before birth do not develop a normal sagittal shape and this area also represents a region of stiffness. In the thoracic region a normal kyphotic shape is essential so that the axis of spinal rotation is anterior to the vertebral bodies in order to protect them from rotation. 36 With block vertebrae the axis is now sited more posteriorly and, as the block is not always symmetrical in the coronal plane, this latter provides directional instability to the block and thus a rotational lordoscoliosis is produced in exactly the same fashion as with idiopathic scoliosis. It is therefore not uncommon to encounter an apparently idiopathic deformity with a disk space that is absent or difficult to visualize to which the semantically incorrect term congenital idiopathic scoliosis would appear very appropriate (▶ Fig. 6.15). As we have seen, if the area of congenital fusion is cervicothoracic and resists rotation locally, then rotation can be translated lower down the spine, as not uncommonly occurs with the Klippel Feil syndrome.
Fig. 6.15 Congenital idiopathic scoliosis. At T3//4 there is a failure of segmentation bilaterally (arrows) and this generates an idiopathic-type curve below.
It is quite clear that not only is the thoracic spine the most common location for progressive anomalies it is also the most common site for progression, in the Minneapolis series 17 of the 28 thoracic curves progressing to a maximum of 93 degrees. 37 In the Edinburgh series hemivertebrae and bars were again particularly prevalent in the thoracic spine: 18 in the thoracic spine and 10 in the thoracolumbar spine. Meanwhile hemivertebrae were common in the lumbar and lumbosacral areas: 19 lumbar and 12 lumbosacral. Of course the reason why there were so many untreated congenital anomalies to study was that nonoperative treatment in the form of casts, casts and braces, or braces alone were, like with idiopathic scoliosis where at best vertebral growth is normal, quite ineffective and the alternative of posterior fusion in situ was at least uncertain and often damaging by adding to the lordotic sagittal plane problem by tethering posterior spinal growth in bone.
In 1986 McMaster published 104 patients with 154 hemivertebrae that had produced scoliotic curves; 65% were fully segmented, 22% semi-segmented, and 12% were incarcerated. 40 Again there were almost twice as many females as males. The hundred diagnosed before skeletal maturity were then followed for a mean of 5 years. The 65% fully segmented hemivertebrae were evenly distributed throughout the spine with equal numbers on each side and were usually triangular in shape with normal disk spaces above and below. These were two relatively normal vertebrae above and below which became slightly wedge-shaped during growth.
The 34 semi-segmented hemivertebrae were most common in the lumbar region and tended to be single. In some cases it wasn’t until after the first year or two of life that ossification showed these hemivertebrae to be semi-segmented.
McMaster concluded that incarcerated and semi-segmented hemivertebrae do not need treatment and fully segmented hemivertebrae may require treatment according to site and number and the stopping of growth on the overgrowing side should be the aim of treatment, but, of course, fusion was the only surgical technique used in those days. 40
Minor degrees of anterior spina bifida can produce the appearance of butterfly vertebra (▶ Fig. 6.8).
Finally, it is becoming more common for patients to be referred prenatally with a hemivertebra visualized on ultrasonography (▶ Fig. 6.16). Wax et al described 19 fetuses with a hemivertebra diagnosis at a mean gestational age of 20 weeks and those fetuses had additional anomalies, often syndromic, that affected prognosis. 45 They were often delivered before term and had either growth restriction or higher mortality rates.
Fig. 6.16 A hemivertebra visualized on a prenatal ultrasound scan.
(With grateful thanks to Dr. Mike Weston, Consultant Radiologist, the Leeds Hospitals.)
Treatment
Back in the Dark Ages(5) the Milwaukee brace was prescribed, unsuccessfully of course, in the nonoperative treatment of congenital scoliosis. 46 Not long after came the results of spinal arthrodesis for congenital spinal deformity in patients younger than 5 years of age. 47 At this time in the late 1970s and even early 1980s the only surgical treatment prescribed by these centers was a big posterior fusion mass to try and stop these deformities from progressing. Of course what a posterior fusion in situ was doing was to try and solidify in bone the preexisting deformity with no correction at all and indeed a distinct possibility of worsening the deformity by posterior bony tether. 48, 49 This was rather like carrying out a posterior fusion for clubfoot. The proponents concluded that early spine fusion for selected difficult deformities appeared to be a highly satisfactory procedure 47 and refuted the experience of Leatherman and Roaf that such fusions would become a posterior tether facilitating further deformation 48, 49 (▶ Fig. 3.16). Thus it was that early posterior fusion in situ with as big a fusion mass as possible became the Holy Grail to be sought by all treating such deformities at that time and still perceived in some quarters as the gold standard.
Meanwhile back in the 1950s, Robert Roaf from Oswestry, England, an orthopaedic intellectual giant of his time, conceived the concept of the treatment of progressive scoliosis by unilateral growth arrest. Most of the 180 patients he reported in 1960 were idiopathic although there were several congenital cases. 49 His main object of treatment was to “correct the disfigurement and hopefully restore a normal appearance.” “Hence over a number of years I have tried to control progressive deformity by inhibiting growth on the convex side.” He added, “for many years I tried posterior spinal fusion but did not succeed in controlling the deformity.” 49 “There are two reasons for this,” he said: “the usual area of posterior fusion for lordoscoliosis with rotation lies in the concavity of the curve and by acting as a tether it accentuates the inhibition of growth and even increases the deformity” and (2) “the new bone of the fusion is no less plastic than the rest of the child’s skeleton so that if the deforming forces are strong it tends to bend with growth.” If learning, and indeed established, spinal deformity surgeons want to understand the importance of the sagittal plane, then Roaf’s papers are a must-read starting point. It is disappointing/somewhat annoying that his hugely important work is not more internationally known.
This led him to the more logical step of combining anterior and posterior convex growth arrest but this of course depended upon there still being growth potential on the concave side, not necessarily the case with congenital scoliosis. Improvement wasn’t great but the majority of his patients improved by up to 20 degrees, 49 a whole lot better than posterior fusion in situ which of course could never lead to curve improvement.
This concept of trying to stop growth on the anteroconvex side of the spine soon caught on and in 1981 Winter reported on 10 children with anterior and posterior hemiarthrodesis with two improvements and the rest nonprogressive. 50 Winter and colleagues went on to report on 13 patients with progressive congenital scoliosis treated by anterior and posterior hemiarthrodesis and hemiepiphysiodesis. 51 They admitted after all that the Holy Grail of early bilateral posterior fusion was not the universal panacea and had mixed results, sometimes arresting progression and at other times, despite a solid fusion the curve progressed, the solid fusion mass bending under the forces of growth just as Roaf had predicted. 49 Curve progression was altered in all cases and two patients showed evidence of improvement but longer follow-up was necessary.
Just as Roaf had found out that the ideal patient had a pure scoliosis, that is, with no significant lordosis, 36 and so with these simple deformities lying only in the coronal plane then clearly stopping growth on the convex side might allow the concave side to catch up. The results were however not reliable and only a few other centers followed suit. 52
Meanwhile Andrew and Piggott reviewed Roaf’s long-term results 53 and found them to be unsatisfactory but this really was because the majority of Roaf’s cases were infantile progressive idiopathic lordoscolioses and not simple hemivertebrae. By the time the logic of the growth arrest procedure could catch up, the biomechanics of the rotational lordosis had overwhelmed the deformity. 36
The fundamental difference between deformities of congenital origin and those that are idiopathic is that the former are rigid from an early stage and this is because there is asymmetric deformation of vertebral shape at the keystone curve apex. Unlike its idiopathic counterpart, there is no inherent slack to be taken up by instrumentation and so the objective of surgery is to address the curve apex. If the problem is of a hemivertebra then excision of this misshapen vertebra suffices but if the anomaly also incorporates failure of segmentation then the whole anomaly can be excised in toto at the apex in wedge shape fashion. If this is not done until the secondary deformity of a long scoliosis is established then the entire structural curve needs to be corrected and fused as Leatherman clearly pointed out 54 (▶ Fig. 6.10). Hence the attraction of dealing with the problem before secondary deformation develops above and below a curve. In this regard hemivertebrectomy at a very young age has become the optimal option.
Although we recognize the pioneering contributions of Royle, 55 Compere, 56 Von Lackum and Smith, 57 and Wiles, 58 the really important advances were made by Roaf in Liverpool 59 and Leatherman in Kentucky. 48 Roaf and Leatherman understood better than anyone else at the time about the three-dimensional nature of structural scoliosis and as regards rigid curves Roaf stated “obviously the rational approach to the problem is to excise a wedge of bone from the apex of the curve” (▶ Fig. 4.10, ▶ Fig. 6.17, and ▶ Fig. 6.18). He likened this to the correction of a pes cavus. It differed from previous descriptions, such as the relatively minor procedure of Royle in excising an accessory hemivertebra, and encompassed apical wedge resection for a rigid deformity of any etiology. However, just like the previous paper it was largely ignored by the major scoliologists at the time who did not seem to read all that widely, at least internationally. It certainly is another must-read paper, that any scoliosis surgeon should study particularly if proposing to carry out an osteotomy.
Fig. 6.17 Our diagram with an apical hemivertebra; the segmental curve is the important one.
Fig. 6.18 (a) First stage of Roaf’s operation: removal of laminae, transverse processes, and vertebral end of two ribs. (b) Exposure of cord, nerve roots, and vertebral bodies and discs. (c) Removal of most of the vertebral bodies and disks and pedicle. (d) Cross section showing the amount of vertebra removed.
(Reproduced with permission and copyright © of the British Editorial Society of Bone and Joint Surgery, Roaf R. Wedge Resection for Scoliosis. J Bone Joint Surg (Br) 1955;1:97–101.)
The American Journal of Bone and Joint Surgery turned down Leatherman’s life’s work (editorial boards have a lot to answer for) and so it was sent to the British Journal of Bone and Joint Surgery who were delighted to accept this distinguished man’s pioneering work and moreover was selected by Professor James (President of the British Orthopaedic Association [B.O.A.] at that time) to be the opening paper of the annual meeting of the B.O.A. in Liverpool in 1977.
Unfortunately for Roaf the only way he could close his wedge was by the use of a corrective plaster jacket as no instrumentation was available at that time. Notwithstanding, excellent corrections were obtained and in one illustrated case in his classic paper a curve was corrected from 70 degrees to 10 degrees! Look at the pictures. In all, 16 cases were described with no complications. Roaf’s vertebral excision was however incomplete in that he left behind the concave third of the vertebra. This of course acted as a hinge so that there wasn’t complete instability at the osteotomy site prior to reduction and plaster jacket application. Being a wedge resection this technique could be applied to deformities other than a single hemivertebra. Moreover, being a closing wedge osteotomy the spinal cord was not unnecessarily stretched.
Then in Hong Kong Hodgson, following his great experience of anterior spinal fusion for tuberculosis, 60 turned his attention to the correction of fixed spinal curves. He favored an opening wedge osteotomy but this stretched the spinal cord at the apex of the deformity and resulted in an unacceptable level of paralysis. 61 In South Africa George Dommisse carried out 68 of Hodgson’s circumferential osteotomies but there were four paraplegias. 62 Meanwhile in Louisville, Kentucky, Leatherman had successfully carried out resection of vertebral bodies anteriorly for years 48 and, unlike Roaf’s this was a complete wedge. But at that stage he had no reliable metalwork, other than staples, to secure his wedge resection after closure. Then with the introduction of Harrington instrumentation, and in particular the Harrington compression system, he now had a method of closing his wedge and securing it rigidly (▶ Fig. 6.19). He then reported all of 67 cases of anterior and posterior wedge resection for rigid spinal curves in 1979 with excellent results. 54 ▶ Fig. 6.20 and ▶ Fig. 6.21 demonstrate Leatherman’s brilliance.
Fig. 6.19 Leatherman’s wedge resection. (a) A wedge has been removed anteriorly leaving behind only the concave pedicle and facet joint; a hemostatic agent fills the gap. (b) The posterior part of the wedge has been removed including the concave facet joint and pedicle and a compression system has been inserted on the convex side. (c) The compression system has been tightened to close the wedge. (d) A distraction rod has been inserted on the concave side to aid correction and stability. A posterolateral spinal fusion with abundant iliac crest bone graft completes the procedure.
Fig. 6.20 (a) This 10-year-old girl with spina bifida with ambulatory ability has a severe congenital bony scoliosis above her spina bifida area and needs a stick to prevent her falling over. (b) PA radiograph demonstrating the severe congenital scoliosis due to mixed anomalies and a marked pelvic tilt. (c) PA radiograph showing a superb correction having taken as much of a wedge as we felt safe. (d) A well balanced spine without pelvic obliquity.
Fig. 6.21 (a) A 4-month-old infant with a severe upper right congenital scoliosis due to the dangerous combination of a unilateral bar on one side and a hemivertebra on the other. (b) No treatment was sought and by the age of 2 the deformity had worsened considerably. (c) Back view of the little boy confirms the severe deformity. (d) After the first stage high thoracotomy and anterior wedge removal. (e) After the second stage there has been a good correction of the deformity and excellent spinal alignment. The compression system on the left has been inserted in distraction mode, a useful modification when you want a small implant. (f) PA radiograph at the age of 8 showing a solid fusion and maintenance of the corrected position. (g) At age 8 the head, neck, and torso are perfectly aligned. No one else was doing this in the late 1960s.
This was revolutionary stuff (unnecessarily radical and potentially dangerous) as the critics were quick to inappropriately point out, at a time when the perceived wisdom was a simple posterior fusion in situ. Leatherman staged his procedure with 1 or 2 weeks between the anterior first and posterior second stages simply because he felt that to do it all at once might be too much for the patient (and the surgeon) but soon one-stage resections were reported. 63 These Leatherman procedures tended to be carried out on established congenital deformities of some magnitude simply because early referral was not common plus the fact that many had had a tethering posterior fusion in situ beforehand. Leatherman already knew that the natural history of a solitary hemivertebra was unpredictable and could cause severe and relentless progression long before others appreciated that 64 (▶ Fig. 6.10). That was why the lower age limit in his series of 60 cases was 2 years and 3 months thus showing Leatherman’s understanding of trying to remove hemivertebrae and so “altering the deforming forces in the growing spine” which indeed was his life’s mission statement.
Of course with the use of anterior and posterior techniques in wedge resection, this mandated an approach through the chest, abdomen, or both and it was not long before there were reports of hemivertebra resection through a single posterior approach. 65– 67 Nakamura started posterior wedge resections in 1984, but he used Harrington instrumentation and in particular the compression system like Leatherman had done to close his wedge. 66 Furthermore these tended to be older children and in Karlsbad our goal was to perform the posterior hemivertebra resection in the first 2 years of life before the deforming effect of the hemivertebra had exerted its secondary damage with growth. In 1991 in Karlsbad we began to put into practice this exciting concept 68 and in 2002 JH reported upon his 21 consecutive hemivertebra cases dealt with by posterior resection, transpedicular instrumentation, and short segment fusion but with a mean age of almost 6 years and the youngest being 1 year and 2 months. All these 21 patients achieved excellent corrections of more than 60%, down to a Cobb angle of 15 degrees or so (▶ Fig. 6.22).
Fig. 6.22 (a) Preoperative radiograph of a 14-month-old girl with a fully segmented hemivertebra, causing a scoliosis of 48 degrees. (b) After resection of the hemivertebra and transpedicular instrumentation with a cervical plate system there is nearly complete correction in the frontal plane. (c) And in the sagittal plane. (d–g) Age 11 years there is maintenance of the corrected position and no visible clinical deformity.
It was clear, in parallel with this clinical experience, that first of all the feasibility of pedicular screw instrumentation in the very young had to be validated. Inherent with this approach would be that surgery would only have to be localized to the primary area of deformation with short segment fixation and fusion. Two particularly important questions arose when considering pedicular screw fixation in 1- or 2-year olds: the first being the size of the pedicles at this age and would they be big enough in the thoracic and lumbar spine to accept a pedicular screw and if so what would be the best screw diameter. 69 Second there might be concerns about vertebral growth after screw insertion and we did not want any vertebral body growth retardation or any spinal canal narrowing. We knew something about the morphometric characteristics of pedicles in young children from the work of Ferree 70 but their youngest child was 3 years of age and only lumbar pedicles were looked at. However, Zindrick had looked at anatomical specimens of immature spines and looked at pedicle morphology from T1 to L5 but again his youngest specimen was already 3 years of age. 71 Ferree’s pedicular diameters ranged from 6 to 12 mm and Zindrick’s from 3 to 8 mm. Of the first 19 consecutive patients whom we reported using pedicle screws in 1- and 2-year-old children, the first 11 were in conjunction with a small plate and rod system (from use in the cervical spine) (▶ Fig. 6.22) but we then developed a new screw/rod system in 1998 and applied it to a further eight children. These were polyaxial titanium screws of diameter 3.5- and 3-mm rods (baby-Moss-Miami) which ideally fitted the dimensions of small children in the thoracic and lumbar spines (▶ Fig. 6.23).
Fig. 6.23 Baby Moss-Miami. (a,b) A 3-month-old child with multiple congenital anomalies of the spine but balanced in the thoracic spine with a midlumbar fully segmented hemivertebra showing rapid progression within the first year. (c,d) Two years after resection of the hemivertebra and instrumentation with a transpedicular screw rod system.
(Reproduced with permission from Lippincott WW. Spine 2002;Vol 27(10):116–1123, Figure 4, p. 1121.)
We found no neurological complications with their use. A monosegmental fusion was performed in all cases. Long-term follow-up of instrumented cases showed no significant retardation of growth in the instrumented vertebrae compared to the adjacent ones and CT showed no major spinal canal stenosis 69 (▶ Fig. 6.24 and ▶ Fig. 6.25).
Fig. 6.24 (a) Hemivertebrectomy at T12/L1. (b–e) Axial MRI scans 11 years later showing no problem with the size of the central canal and therefore no spinal cord problems.
(Reproduced with permission from Lippincott WW. Spine 2002;Vol 17:1791–1799, Figures 5 and 7.)
Fig. 6.25 Axial CT scan 8 years after hemivertebrectomy at L2/3 showing normal pedicular and vertebral growth 8 years postoperatively.
We then reported posterior hemivertebra resection with transpedicular instrumentation in 28 children aged 1 to 6 years with 12 thoracic cases, 12 thoracolumbar, and 4 lumbar. 72 In eight cases there was an associated unilateral bar that was divided after hemivertebra resection (▶ Fig. 6.26). There were no neurological complications and no significant growth retardation of the pedicles on scanning and nor was there any evidence of spinal canal stenosis. In two patients with severe pseudokyphosis a convex pedicle was overloaded and broke requiring temporary one level additional segment instrumentation and two patients required further surgery—one because of bar formation at the operative site and one because of a new bone mass at the site of the resected hemivertebra. All patients had an excellent final clinical result with a Cobb angle of 45 degrees reduced to 13 degrees after 3.5 years of follow-up. Complete correction of the local deformity with a short segment fusion allows for normal growth of the unaffected parts of the spine. 72
Fig. 6.26 (a–c) Double hemivertebrae with a contralateral bar in a 3-year-old girl; worst case scenario regarding prognosis. (d,e) After hemivertebra resection and division of the bar a good correction was obtained. (f) Four years later the correction is maintained and there is a solid fusion.
Others were tackling the problem of hemivertebrae at an early age somewhat differently. In 2005 the Boston group reported on 18 children under the age of 8 with a congenital spine deformity who had been treated with reduced size instrumentation and short segment fusion with excellent and safe curve correction with a minimum of 3 years of follow-up. 73 This was however done through both an anterior and posterior combined approach as did Xu in China. 74
Garrido et al performed a short anterior instrumented fusion along with a posteroconvex noninstrumented fusion 75 while Elsabaie et al carried out an anterior single stage partial vertebrectomy with instrumentation reporting good results in 12 children with a mean age of just less than 3 years with corrections from 48 degrees down to 17 degrees. 76 The advantages of a one-stage posterior hemivertebra resection with transpedicular instrumentation were also noted in China with Yu from Nanjing reporting on 27 consecutive cases with a mean age of 5.5 years but with an average of more than four segments. 77 Then Peng from Guangzhu reported on 10 patients under the age of 5 with a unilateral posterior approach with a single rod and pedicle screws. At follow-up averaging 3.5 years there was a 63% segmental curve angle correction (▶ Fig. 4.10 and ▶ Fig. 6.17). It was recommended as less traumatic, simple, and safe. 78
In 2009 Lenke et al reported on 35 patients treated by posterior only vertebral column resection (VCR) carried out between 2000 and 2005 for both congenital scoliosis and kyphosis producing what they described as “dramatic radiographic and clinical corrections.” 79 Perioperative spinal cord monitoring was regarded as being mandatory as there were two temporary monitoring problems that reverted to normal after “prompt surgical intervention.” There were several different patient categories including both severe scoliosis and kyphosis but the average patient age was beyond 10 years therefore not focusing on the very young.
Then in 2011 a multicenter comparison of three surgical techniques was reported by Yasnay et al. 80 Hemivertebra resection with instrumented fusion was compared with instrumented fusion without hemivertebra resection or hemiepiphysiodesis or in situ fusion. Seventy-six patients were studied in all with a mean age of 8 years. The instrumented fusion with hemivertebra excision group were youngest (mean age 5 years) but with a much better correction at 73%. However, there was a higher neurological complication rate although all such patients made a complete neurological recovery. There was also a higher instrumentation problem rate requiring revision surgery.
Then in 2009 we published our experience of 41 very young children (mean age 3.5 years) followed up for more than 6 years. Group 1 comprised 28 children with hemivertebrae only who underwent posterior hemivertebra resection and pedicular instrumentation while group 2 comprised 13 children with a contralateral bar who required hemivertebra resection plus additional osteotomy of the bar and/or rib fusions. In group 1 the main (segmental) curve was reduced from 36 to 7 degrees while in group 2, with bigger initial curves, the main curve reduced from 70 degrees to 23 degrees. 81
Thus it can be seen that apical wedge resection carried out from a purely posterior approach with the use of pedicular instrumentation can be utilized to deal with not only simple solitary hemivertebrae but also more complex deformities including those with concave bar formation (▶ Fig. 6.26) or multiple hemivertebrae (▶ Fig. 6.27). This case shows the importance of checking on the sagittal plane after posterior instrumentation. We only fuse the area of the congenital deformity and so the posterior instrumentation covers several segments above and below so as to control the deformity in the frontal plane. The posterior instrumentation does however stop spinal growth at the back of the spine and so continuing anterior growth can cause an increasing thoracic lordosis that will hinder correction in the frontal plane. Moreover, if the lordosis is unchecked, patients find the displeasing cosmetic effect just as bad as a rib hump. Controlling growth of the structural curve above and below the area of the congenital anomaly which we have fused is extremely important, particularly during the preadolescent/adolescent phase of increased growth acceleration. When this period has been safely negotiated then the whole structural curve must be fused, otherwise there will be a situation of a mobile deformity versus rigid metalwork that is bound to lead to ultimate metalwork breakage or loosening. Therefore, it is important that these patients are followed up until spinal maturity.
Fig. 6.27 (a–c) Reformatted 3D CT scans showing multiple thoracic hemivertebrae: one on the right at T4, one on the left at T8, and again on the left at T11. (d,e) PA and lateral radiographs showing 80 degree left thoracic curve with significant rotation (note the rib asymmetry). Also note the apical lordosis on the lateral view. (f,g) PA and lateral radiographs after resection of the hemivertebrae and instrumentation, the spine is straight in the frontal plane and a natural kyphosis in the lateral plane.
(h,i) PA and lateral radiographs three years after surgery showing that a natural kyphosis that was present immediately after surgery has developed into a thoracic lordosis. The posterior metalwork has restricted posterior growth and allowed the front of the spine to continue. (j,k) PA and lateral radiographs after removal of the rods but we left the screws. (l,m) PA and lateral radiographs after insertion of the right rod just to control the frontal plane while the lateral radiograph shows restoration of a normal kyphosis.
(n) PA radiograph after insertion of the second rod. (o) Composite lateral radiographs showing initial kyphosis followed by the development of a significant lordosis and then restoration of a normal thoracic kyphosis.
To reiterate, the important concept of early apical resection is to remove the deforming force before it creates either a primary local scoliosis or any secondary scoliogenic effect elsewhere in the spine (▶ Fig. 6.10 and ▶ Fig. 6.14). Thus many of these potentially difficult deformities can be dealt with by a one-level localized short segment spinal fusion in the very young. Don’t wait and see what happens.
Congenital anomalies in the neck and cervicothoracic junction require special attention if they are to be dealt with surgically. Obviously the pathological bony deformity should be clearly established by whatever imaging techniques. Then, when there are congenital bony anomalies there is an increased incidence of associated vascular anomalies with particular reference to the vertebral arteries. ▶ Fig. 6.28 shows the normal arterial anatomy from the root of the neck upward. The vertebral artery normally arises from the first part of the subclavian artery and then passes upward through the transverse process foramina from the sixth cervical vertebra upward. The vertebral artery may however enter the transverse foramina at the fourth, fifth, or seventh cervical vertebrae and may itself arise from the common carotid artery rather than the subclavian. It is mandatory therefore to perform angiography/arteriography to determine the arterial situation prior to surgery (▶ Fig. 6.29). Furthermore, you have to anticipate that there is a risk of damage to the vertebral artery and so you have to know whether the contralateral vertebral artery can take over the blood supply to the brain. For this purpose, an occlusion test is performed bilaterally. A vascular catheter has to be inserted into the vertebral artery on one side and by opening a balloon at the tip of the catheter you perform a temporary occlusion of the artery. Then you have to observe the patient for 5 to 10 minutes to determine whether a neurological deficit occurs. Then you know perfectly whether the contralateral artery is sufficient enough to provide the blood supply of the brain (▶ Fig. 6.30). Without performing this occlusion test you should never resect a hemivertebra in the cervical spine or the cervicothoracic junction. Once this has been done then the vertebral resection can be confidently carried out.
Fig. 6.28 Vascular anatomy.
(Reproduced with permission from Clinical Anatomy, 7th edition, Ed Snell RS, Lippincott, Williams and Wilkins, 2004, Figure 11.12, p. 737.)
Fig. 6.29 (a) Full length spine film showing a hemivertebra on the left at C6. There is also an anomaly in the upper-mid thoracic spine. (b) PA 3D CT scan of the upper spine very nicely showing the hemivertebra at C6 on the left. (c) Lateral 3D CT view showing that two of the upper thoracic vertebrae are fused together with resultant kyphosis. (d) AP frontal angiography CT showing the vertebral arteries entering the transverse process foramen through C6 but being a little compromised at C7 particularly on the left. (e) A balloon-catheter has been inserted into the left vertebral artery and an occlusion has been performed before it forms the basilar artery with its namesake on the right side. (f) At the same time there is also a catheter in the right vertebral artery and during the occlusion of the left vertebral artery contrast medium is injected into the right vertebral artery; the blood flow demonstrates that there is a perfect blood supply of the brain. Meanwhile the patient is checked clinically and by spinal cord monitoring (SCPs and MEPs). Therefore, we know very precisely if the potential damage to the left vertebral artery will be a problem for the patient.
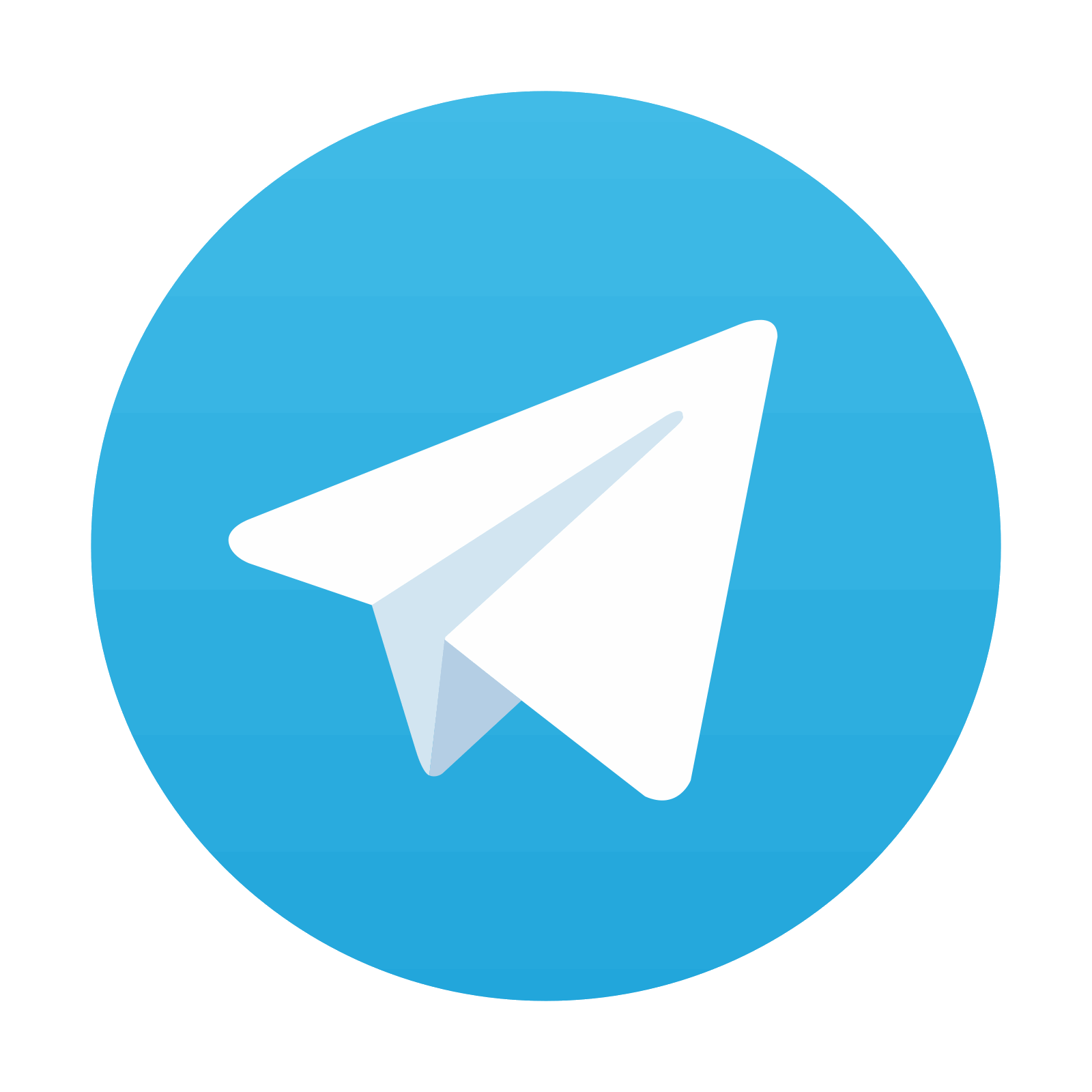
Stay updated, free articles. Join our Telegram channel
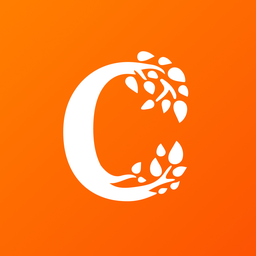
Full access? Get Clinical Tree
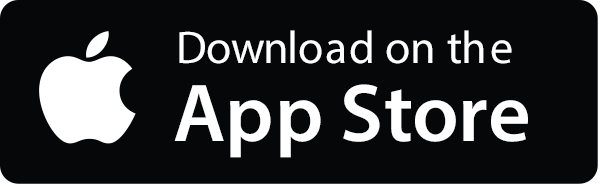
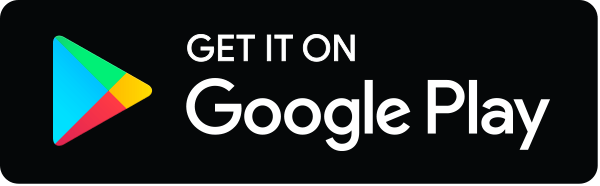