Thiviya Selvanathan, Mike Seed, Vann Chau Congenital heart disease (CHD) is the most common birth defect and occurs in approximately 1% of live births.1–5 A recent systematic review and meta-analysis showed an increase in the birth prevalence of CHD globally from 1970 to 2017 with the highest prevalence seen in Asia (9.3 per 1000 live births) and lowest in Africa (2.3 per 1000 live births).2 This increase was mainly driven by a change in the prevalence of mild CHD lesions (e.g., atrial septal defect, ventricular septal defect, patent ductus arteriosus) during the study period, likely reflecting improvements in the screening and detection of these lesions over time, although other studies have not observed a similar increase in prevalence.1,6 This variation may be due to differences in definitions of CHD and study periods that were included across studies. Approximately one-third of infants with CHD have severe malformations requiring surgical interventions within the first year of life. This was previously associated with significant mortality, however, with advances in cardiac surgical and intensive care, there has been an increase in the survival of infants with CHD with now >85% surviving into adulthood.1,7,8 This has led to an increase in the lifetime prevalence of CHD, and >65% of the entire CHD population are adults.9 As a result, there has been an expanding body of research focused on understanding long-term outcomes in patients with CHD. Neurodevelopmental impairments are common in severe CHD; they are present in over 50% of children.10 The typical neurodevelopmental profile of children with CHD consists of mild but highly prevalent deficits across multiple domains: visual-spatial skills, executive function, memory, language, motor skills, social interactions, and behavior.10–12 Although IQ scores in children with CHD on a group level are typically within the normal range, they are significantly lower when compared to population normative data or healthy control children.13–18 Neurodevelopmental impairments emerge early in childhood with predominantly motor delays seen in infants with CHD.19,20 However, as children with CHD become older, abnormalities in cognition, adaptive skills, and behavior become more apparent as cognitive and social expectations change with increasing age.13,21–25 This highlights the importance of long-term neurodevelopmental follow-up in this population. These cognitive impairments persist throughout adolescence and adulthood.16,18,26–30 Interestingly, a recent cohort study observed an increased risk of dementia, particularly early-onset dementia, in CHD adults compared to the general population, with the highest risk seen in severe CHD.31 Adults with CHD are also more likely to be unemployed and achieve lower levels of education.32–34 Further longitudinal studies following patients into late adulthood are needed to understand long-term neurological outcomes and psychosocial functioning in individuals with CHD. Although mild, the highly prevalent neurodevelopmental and cognitive abnormalities in CHD have important functional consequences. Children and adolescents with CHD have an increased need for educational support, and lower employment rates are seen in adults with CHD.10,15,35 Understanding the biologic basis of neurodevelopmental abnormalities in CHD and their key contributing factors are critical in developing effective interventions and management strategies that support optimal neurodevelopmental and cognitive outcomes. Neuroimaging studies have observed that brain dysmaturation, which begins antenatally, and brain injury are the key brain changes that underlie adverse neurodevelopmental outcomes in CHD. Several risk factors for neurodevelopmental impairments in CHD have been identified and include innate (genetic) as well as acquired and potentially modifiable (prenatal diagnosis, perioperative management, socioeconomic status [SES]) factors. This chapter reviews abnormalities in brain maturation and common types of brain injury observed in infants with CHD, as well as key contributors to these brain changes. Neuromonitoring and neuroprotective strategies that are currently in use or are under investigation to promote optimal brain health and neurodevelopment in CHD are also discussed. Finally, we highlight key knowledge gaps and areas in need of further study to improve neurodevelopmental outcomes in this population. The etiology of CHD is multifactorial with both environmental and genetic predisposing factors as shown in Fig. 16.1. Environmental contributors to CHD include antenatal exposures to infections (e.g., rubella),36 teratogens (e.g., retinoic acid, phenytoin, lithium),37 and maternal chronic conditions (e.g., obesity, diabetes mellitus, phenylketonuria).38–41 The genetics of CHD is complex and heterogeneous; both inherited and de novo genetic alterations cause CHD.42 Several genetic syndromes have been associated with CHD including aneuploidy syndromes (e.g., Down syndrome or trisomy 21), copy number variants (e.g., DiGeorge syndrome or 22q11.2 deletion), and single gene mutations (e.g., Noonan syndrome).43,44 With advances in genetic and genomic technologies, recent studies have reported de novo single nucleotide variants across hundreds of genes involving multiple biological pathways that contribute to CHD, including in isolated (nonsyndromic) CHD.45–47 Moreover, recent studies have shown gene-environment interactions between Notch signaling and maternal hyperglycemia and hypoxia resulting in an increased incidence of CHD in mice.48,49 The complex interactions between environmental and genetic risk factors for CHD are an area that warrants further study. Severe CHD is often categorized as single ventricle or biventricular lesions, and may be associated with aortic arch obstruction such as aortic coarctation and aortic valve atresia or stenosis. Severe CHD also frequently includes intracardiac (e.g., atrial septal defect or ventricular septal defect) or extracardiac (e.g., patent ductus arteriosus) shunts. The presence of shunts, along with abnormal cardiac connections, malformed valves, and obstructions to blood flow may result in reduced systemic oxygen delivery due to the mixing of venous and arterial blood. Two common forms of severe CHD accounting for a large proportion of surgeries performed in the neonatal period are transposition of the great arteries (TGA) and patients with single ventricle physiology, including those with hypoplastic left heart syndrome (HLHS), with much of the literature of neurodevelopment, brain maturation, and brain injury in infants with CHD focusing on these two high-risk populations. TGA (Fig. 16.2) results from ventriculoarterial discordance when the aorta arises from the right ventricle and the pulmonary artery from the left ventricle.50 This leads to the systemic circulation, being supplied with deoxygenated venous blood returning from the body, and the pulmonary circulation, being supplied with oxygenated blood returning from the lungs, to be in parallel rather than in series as they are in a normal heart. This results in the affected infant being cyanosed. TGA can be associated with other cardiac abnormalities, such as ventricular septal defects, left ventricular outflow tract obstruction, or coarctation of the aorta.50 TGA can be difficult to diagnose antenatally with ultrasound, while affected infants can present with cyanosis and tachypnea. The severity of symptoms depends on the presence of other cardiac anomalies and the degree of mixing between the two parallel circulations. The initial management of TGA is to stabilize the infant until the corrective surgery is performed. This includes maintaining the patency of the ductus arteriorus using prostaglandin E1 infusion to optimize circulatory mixing.51 Balloon atrial septostomy may also be performed to improve oxygenation and survival in neonates with d-TGA.52 In this intervention, a balloon is passed into the left atrium, inflated, and pulled vigorously across the atrial septum to create a larger atrial septal defect. The arterial switch operation is now the standard corrective procedure for TGA.53 HLHS (Fig. 16.2) describes a spectrum of cardiac malformations characterized by underdevelopment of the left heart with normally related great arteries, leaving the right ventricle to perfuse both the pulmonary and systemic circulations. In HLHS, there is significant hypoplasia of the left ventricle which is associated with atresia, stenosis, or hypoplasia of the aortic and/or mitral valves, and hypoplasia of the ascending aorta and arch. The anatomic spectrum varies from almost complete absence of left ventricle combined with aortic and mitral atresia to milder hypoplasia of the left ventricle combined with aortic and mitral valve hypoplasia but without stenosis or atresia.54 Survival is dependent on a patent ductus arteriosus and nonrestrictive atrial septal defect to ensure adequate systemic perfusion and mixing of oxygenated and deoxygenated blood. Affected infants become symptomatic when the ductus arteriosus closes and pulmonary vascular resistance decreases as expected after birth, progressing to cardiogenic shock and respiratory failure. Infants with a restrictive or intact atrial septal defect present with severe cyanosis and respiratory distress at birth because of pulmonary blood flow. Fortunately, a prenatal diagnosis is made in approximately 50% to 75% of cases with routine obstetrical ultrasound, typically between 18 and 24 weeks gestation, and is associated with improved survival and decreased morbidity.55–58 The initial management of infants with HLHS is focused on ensuring adequate systemic perfusion, which is achieved with intravenous prostaglandin E1 infusion to maintain patency of the ductus arteriosus. Balloon atrial septostomy may also be used in patients with a restrictive or intact atrial septum. The surgical palliation approach usually consists of a three-staged approach: (1) Norwood procedure (neonatal period), (2) bidirectional Glenn procedure (around 3–6 months of age), and (3) Fontan procedure (typically 2–5 years of age). In the Norwood procedure, a neoaorta is created by using the proximal pulmonary artery and homograft material, which is then connected to the native ascending aorta. A source of pulmonary blood flow is established either via a right ventricle to pulmonary artery conduit (Sano) or by connecting the innominate artery with the proximal right pulmonary artery via a Gore Tex tube (called modified Blalock-Taussig shunt). By 6 months of age, the arterial shunt can usually be substituted with a bidirectional cavo-pulmonary shunt (SVC to pulmonary artery anastomosis), which results in diminished volume loading for the ventricle. As the child grows further, the Fontan circulation can be completed. In this third stage, the inferior vena cava is connected to the pulmonary arteries, allowing the entire systemic venous return to pass through the lungs, driven by the suction force of the heart and finally achieving near normal arterial oxygen saturations. Table 16.1 summarizes common neuroimaging and neuromonitoring studies performed in CHD infants and children, their utility, and expected findings. Abnormalities in brain maturation and growth are common brain changes seen in CHD and are key contributors to adverse neurodevelopmental outcomes. Neonates with severe CHD have preoperative abnormalities in brain microstructural and metabolic maturation when compared to healthy controls.64,72 Smaller head circumferences,73 decreased total and regional brain volumes,74–76 reduced cortical folding,77,78 and alterations in structural and functional brain network connectivity65,66,79 are also present at birth, even before neonates with CHD undergo surgery (Table 16.2). Some studies have observed a link between brain dysmaturation in the neonatal period and adverse neurodevelopmental outcomes later in childhood in CHD, although additional studies are required to further elucidate this relationship.80–82 These alterations in brain maturation persist through childhood and adolescence and are also associated in long-term neurodevelopmental outcomes in CHD.27,83–87 Recent studies have observed smaller brain volumes and altered white matter microstructure in adults with CHD, which are associated with cognitive function.26,88 Table 16.3 summarizes a selected list of studies that have observed associations between brain abnormalities and neurodevelopment and cognition in CHD. Lynch et al. (2021)89 MRI, diffuse optical spectroscopy, diffuse correlation spectroscopy Cerebral oxygen extraction during DHCA Larger decreases in cerebral oxygen saturation during DHCA associated with new postoperative WMI (p = 0.02). Peyvandi et al. (2021)90 Fetal MRI, neonatal MRI CHD lesion type Lower brain volumes with more severe WMI in TGA (p = 0.04) but not HLHS. Schlatterer et al. (2021)91 MRI Autonomic dysfunction Lower autonomic tone associated with preoperative brain injury (p < 0.01). Feldmann et al. (2020)65 MRI, DTI, tractography — Reduced pre- and postoperative global network efficiency in CHD. Larger WMI volume associated with lower network strength and global efficiency. Ng et al. (2020)75 — Volume reduction in basal ganglia, thalami, corpus callosum, and cortical regions, and volume expansion in CSF in CHD. Ni Bhroin et al. (2020)79 MRI, DTI, tractography — Reduced structural connectivity in a cortico-striatal-thalamic subnetwork in CHD. Claessens et al. (2019)72 MRI, DTI SVP Increased preoperative fractional anisotropy in TGA and mean diffusivity highest in SVP. Kelly et al. (2019)92 MRI, NODDI Impaired cerebral oxygen delivery Increased cortical fractional anisotropy and reduced orientation dispersion index in CHD. Kelly et al. (2019)93 MRI BAS Preoperative brain injury in 39%. Strokes (4%) were only seen in patients who had BAS. De Asis-Cruz et al. (2018)66 fMRI — Intact global network topology but reduced preoperative regional functional connectivity involving subcortical areas and brainstem. Peyvandi et al. (2018)94 MRI HLHS More postoperative (p = 0.03) and severe (p = 0.01) brain injury in HLHS compared with TGA. Slower rate of brain growth in patients with severe brain injury (p < 0.01) and HLHS (p < 0.001). Schmithorst et al. (2018)95 MRI, DTI, tractography — Reduced global network efficiency and nodal efficiency in CHD pre- and postoperatively. Fogel et al. (2017)96 MRI Surgical stage More WMI post-BDG (OR 3.68) and post-Fontan (OR 2.0) compared to pre-BDG. Most focal tissue loss post-BDG (OR 8.75) and post-Fontan (OR 6.16) compared to pre-BDG. Peyvandi et al. (2016)97 MRI, DTI Postnatal diagnosis of CHD More brain injury with postnatal diagnosis (p = 0.003). von Rhein et al. (2015)74 MRI — Lower total brain and regional volumes in CHD (p < 0.001). Andropoulos et al. (2010)98 MRI, NIRS SVP Miller et al. (2007)64 MRI, MRS, DTI — Lower preoperative NAA:choline (p < 0.01) and white matter fractional anisotropy (p < 0.001) in CHD. McQuillen et al. (2006)99 MRI BAS 41% had focal preoperative brain injury, associated with BAS (number needed to harm 1.6). Mahle et al. (2002)100 MRI, MRS — WMI: preoperative 16%, postoperative 42% Stroke: preoperative 8%, postoperative 19% Elevated lactate in 53%, correlated with preoperative lesions (p < 0.02) Bonthrone et al. (2021)101 Bayley-III at 22 months MRI Cognitively stimulating parenting associated with cognitive outcomes at 2 years. Ehrler et al. (2021)88 Extensive test battery MRI, DTI Lower fractional anisotropy in CHD adults. Lower executive function scores in CHD adults, associated with lower fractional anisotropy. Kuhn et al. (2021)102 PSOM, Glasgow Outcome Scale-Extended (Pediatric version) between 5 and 23 months of age MRI Longer stay in ICU associated with brain injury (p < 0.001). Stegeman et al. (2021)80 Bayley-III at 3, 6, 18 months. MRI WMI associated with worse gross motor outcomes (p < 0.05). Cortical gray matter and cerebellar volumes (p < 0.05) associated with fine motor outcomes. Repeated cardiac surgery associated with poorer motor outcomes. Verrall et al. (2021)26 Adolescent and adult Fontan (n = 107), controls (TGA, healthy controls) Cogstate battery MRI Fontan group had smaller brain volumes, which were associated with resting oxygen saturations. Noorani et al. (2020)83 MoCA, Wide Range Assessment of Memory and Learning-2 MRI Smaller caudate volumes in SVP. Cabrera-Mino et al. (2020)84 MoCA, Wide Range Assessment of Memory and Learning-2 MRI Smaller mammillary body volumes in SVP. Ehrler et al. (2020)27 Weschler Intelligence Scale for Children-IV MRI, DTI Lower fractional anisotropy in CHD. Lower working memory scores in CHD, which were associated with frontal lobe fractional anisotropy. Hottinger et al. (2020)103 Bayley-III at 1 year MRI Lower pre- (p = 0.01) and postoperative (p = 0.03) brain maturation scores in CHD. Brain maturation not associated with Bayley-III scores. Morton et al. (2020)85 Weschler Intelligence Scale for Children-IV, Delis-Kaplan Executive Function System MRI Differences in sulcal patterns in CHD that are associated with cognitive outcomes. Lim et al. (2019)104 Bayley-III at 18 months MRI Older age at repair associated with lower language outcomes (p < 0.01). Meuwly et al. (2019)105 Bayley-III at 12 months MRI Smaller brain volumes in CHD (p < 0.01). Lower cognitive and motor scores in CHD (p < 0.001). Postoperative brain volumes associated with cognitive and language scores (p < 0.04). Claessens et al. (2018)82 Prospective cohort CHD neonates with aortic arch obstruction (n = 34) Bayley-III at 2 years, WPPSI at 6 years MRI WMI associated with lower cognitive scores (p < 0.05). Injury to posterior limb of internal capsule associated with motor outcomes (p = 0.03). Smaller basal ganglia and brainstem volumes associated with lower IQ (p = 0.03). Peyvandi et al. (2018)106 Bayley-II at 12 and 30 months MRI WMI associated with motor outcomes at 30 months (p ≤ 0.05). Watson et al. (2018)86 Fontan children and adolescents Weschler Intelligence Scale for Children, Weschler Adult Intelligence Scale MRI, DTI Fractional anisotropy correlated positively with processing speed and full-scale IQ. Rollins et al. (2017)107 Bayley-II, MacArthur-Bates Communicative Development Inventories (CDI) at 1 year MRI Smaller total and regional brain volumes in CHD (p < 0.01). Brain volumes correlated with CDI language scores (p < 0.05). Brain dysmaturation in CHD begins during fetal brain development (Table 16.4). A fetal neuroimaging study of fetuses with CHD observed smaller total brain volumes and lower NAA:choline ratios (reflective of metabolic maturation) compared to controls. In this study, the differences in metabolic maturation and brain volumes between CHD and control fetuses widened over the third trimester during a period when brain growth and maturation is expected to accelerate, suggesting that the impairments in brain maturation observed in fetuses with CHD progress throughout gestation.62 Fetuses with CHD also have smaller regional brain volumes,108 slower head growth,109,110 and delayed cortical development.63,111 Interestingly, fetuses with CHD had less pronounced reductions in regional brain volumes when compared to fetuses with a family history of CHD rather than when compared to control fetuses with no family history of CHD, suggesting that genetic or shared environmental factors may contribute to brain dysmaturation in fetuses with CHD.108 Paladini et al. (2021)112 Case-control CHD (101/522) Fetal ultrasound Smaller frontal lobe anteroposterior diameter/occipitofrontal diameter ratio (p < 0.001) in CHD fetuses. Peyvandi et al. (2021)90 Prospective cohort of TGA (n = 37) and HLHS (n = 26) Fetal MRI, neonatal preoperative MRI Lower total brain volume (fetal and neonatal scans) associated with increased risk of postnatal moderate-severe WMI in TGA, but not HLHS. Ren et al. (2021)113 Case-control CHD (40/160) Fetal MRI Smaller gray matter, subcortical brain tissue, cerebellar and brainstem volumes and larger CSF and ventricular volumes in CHD fetuses. Ren et al. (2021)114 Case-control CHD (50/150) Fetal MRI, DWI ADC values lower in frontal and periventricular white matter and pons and higher in thalamus in CHD fetuses. Rollins et al. (2021)108 Case-control CHD: HLHS/TGA (24/179), other CHD (50/179) Fetal MRI Smaller brain volumes in HLHS/TGA fetuses (p < 0.001). Smaller subplate and intermediate zone volumes (p < 0.01) in HLHS/TGA fetuses. Inversetti et al. (2020)109 Case-control CHD (79/229) Fetal ultrasound Lower head circumference in CHD fetuses after second trimester (p < 0.01). Fetuses with cyanotic CHD had slower head growth than acyanotic CHD (p < 0.05). Jaimes et al. (2020)115 Case-control CHD (48/69) Fetal MRI Lower fetal total (brain) maturation score (p < 0.01) in CHD fetuses. Wu et al. (2020)116 Case-control CHD (48/140) Fetal MRI Maternal psychological distress associated with smaller hippocampal and cerebellar volumes (p < 0.05) in CHD fetuses. Claessens et al. (2019)117 Prospective cohort CHD (n = 61) Fetal MRI, neonatal pre- and postoperative MRI Larger fetal brain volumes correlated with larger neonatal brain volumes. Smaller fetal brain volumes associated with neonatal ischemic brain injury. Ortinau et al. (2019)111 Case-control CHD (17/36) Fetal MRI Altered global sulcation pattern of left hemisphere of CHD fetuses. Olshaker et al. (2018)118 Retrospective CHD cohort (n = 46) Fetal MRI Smaller cerebellar volumes in CHD compared to population norm (p < 0.05) Rajagopalan et al. (2018)119 Prospective cohort of biventricular (n = 7) and single ventricle (n = 10) CHD Fetal MRI Slower cerebral regional (p < 0.05) and cerebellar growth (p < 0.01) trajectories in single ventricle fetuses. Ruiz et al. (2017)110 Prospective cohort CHD (n = 119) Fetal ultrasound Smaller biparietal diameter and head circumference throughout gestation compared to normative data. Wong et al. (2017)120 Case-control CHD (11/62) Fetal MRI, neonatal pre- and postoperative MRI Delayed internal closure of bilateral opercula, enlargement of bilateral lateral ventricles, and smaller cerebellar vermis height in CHD fetuses and neonates (all p < 0.05). Masoller et al. (2016)121 Case-control CHD (58/116) Fetal MRI, MRS, fetoplacental Doppler ultrasound Lower NAA/choline values in basal ganglia and frontal lobe (p < 0.05) in CHD fetuses. Sun et al. (2015)122 Case-control CHD (30/60) Fetal MRI Smaller total brain volume correlated with reduced cerebral oxygen delivery and consumption in CHD (p < 0.001). Clouchoux et al. (2013)63 Case-control HLHS (18/48) Fetal MRI Smaller gyrification index and cortical surface area (p < 0.001). Limperopoulos et al. (2010)62 Case-control CHD (55/105) Fetal MRI, MRS Lower total brain volumes (p < 0.001) and NAA/choline (p < 0.001) in CHD fetuses
Chapter 16: Congenital heart disease: An important cause of brain injury and dysmaturation
Key points
Introduction
CHD etiology and malformation grouping
Transposition of the great arteries
Hypoplastic left heart syndrome
Brain dysmaturation and brain injury in CHD
Brain dysmaturation begins in utero in CHD
Study: First Author (Year)
Study Design
Neuroimaging Modality
Risk Factors
Brain Abnormalities
Study: First Author (Year)
Study Design
Assessment Modality
Neuroimaging Modality
Key Findings
Study: First Author (Year)
Study Design
Neuroimaging Modality
Brain Abnormalities
Stay updated, free articles. Join our Telegram channel
Full access? Get Clinical Tree
Congenital heart disease: An important cause of brain injury and dysmaturation
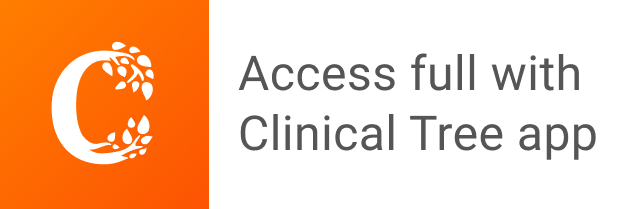