(1)
Nuffield Department of Surgical Sciences, Oxford University, Oxford, UK
Preamble
This tutorial has been written by Dr. Piers Nye, a physiologist who teaches this topic on the Oxford course. It aims to provide an overview of the physiological mechanisms that control cerebral blood flow and explain the brain’s ability to regulate its own blood supply. It is intended to remind students of the basic mechanisms controlling the blood supply to an organ and to cover those that are unique to the cerebral circulation in the healthy individual. An understanding of the factors that control cerebral perfusion is essential for the interpretation of functional imaging studies, such as magnetic resonance images (f-MRI) of the brain and for the development of treatments for neurological disorders such as stroke and Alzheimer’s disease [1]. But the focus of this tutorial is the physiological control of cerebral blood flow, and the effects of pathological interruptions in flow will not be considered further here. It has recently become apparent that, although CO2 (or the associated fall in pH) is an exceptionally powerful determinant of CBF, it is not normally responsible for the tight coupling of flow to neuronal activity. This is done by feedforward mechanisms that depend primarily upon the secretion of neurotransmitters such as glutamate.
5.1 Cerebral Intolerance of Hypoperfusion
Of all the tissues in the body, the brain is the most vulnerable to hypoperfusion. Ischaemic changes and infarction (cell death due to low oxygen) occur within minutes of an interruption of blood flow. This fragility is due to its exclusive aerobic metabolism and the very high metabolic rate required to restore the ionic gradients that are altered by the constant activity of synapses and action potentials. One expression of the remarkable intensity of cerebral metabolism is that though the brain represents only 2% of total body weight, it uses 20% of the oxygen supply. The cerebral blood flow (CBF) that supplies this demand for oxygen comprises 18% of cardiac output or about 50 ml/100 g of brain/min. The brain’s fragility is evident by the loss of synaptic transmission within minutes of flow falling to one-third of its normal value. At one-fifth normal flow (10 ml/100 g/min), membrane pumps fail and neurons and glia die. However, irreversible damage by infarction is not immediate; it takes 10 min to develop at zero flow and about 2 h at 12 ml/100 g/min [2].
5.1.1 Cerebral Protection of Blood Flow
Several mechanisms protect the brain from hypoperfusion injury. The first level of protection is anatomical and is provided by the multiplicity of supply arteries. The paired internal carotid and vertebral arteries anastomose at the circle of Willis to which 80% of CBF comes from the carotid arteries, while anastomoses by some cortical arteries also provide collateral blood flow. The second level of protection is physiological, matching local perfusion to local metabolism by changing the resistance of vessels. The combined effect of these local control mechanisms is to hold CBF stable over a wide range of arterial blood pressure values (Fig. 5.1).


Fig. 5.1
Cerebral blood flow is almost completely independent of perfusion pressure (arterial–venous pressure) between 60 and 150 mmHg. This is shown here by a vascularly isolated brain in which the arterial reservoir is raised to take perfusion pressure from 30 to 200 mmHg. The almost constant blood flow in the normal range of pressures, termed autoregulation, is an intrinsic property of the blood vessels that is independent of the innervation of vascular smooth muscle (VSM) by autonomic nerves
5.1.2 Vascular Resistance
There are only two factors that could possibly determine the rate of flow through the brain and these are the pressure gradient from arteries to veins, i.e. arterial blood pressure (ABP) − venous blood pressure (VBP) and the resistance to flow provided by the brain’s blood vessels, i.e. cerebrovascular resistance (CVR).


ABP − VBP may be abbreviated to cerebral perfusion pressure (CPP) and CVR may be expanded to reveal its components (η = blood viscosity, l = vessel length and r = vessel radius). This relationship was first formulated by Jean Louis Marie Poiseuille1 in 1840 and is therefore known as Poiseuille’s Law [3]. It is directly analogous to Ohm’s Law (I = V/R):


CPP is generally held fairly constant by the arterial baroreflex and it affects all parts of the brain equally, so vascular resistance, which is highly variable and which can increase in one place while it decreases in another, is by far the most important determinant of flow. Furthermore, among the three factors that contribute to resistance, only vessel radius is adjusted physiologically, and because it is raised to the fourth power, a modest change in radius has a very large effect on resistance. A vessel’s smooth muscle need only constrict to half its radius to increase resistance by 2 × 2 × 2 × 2 = 16-fold and, if a vessel were to double its radius, flow through it would increase by 16-fold. If such an increase in flow were to be achieved by changing perfusion pressure, ABP would have to rise from 100 to 1600 mmHg.
5.2 The Blood–Brain Barrier
The blood–brain barrier (BBB) is composed of endothelial cells (ECs), pericytes, astrocyte end-feet and neurons which together make up the ‘capillary neurovascular unit’ [4] (Fig. 5.2). In pre- and post-capillary vessels, pericytes are replaced by vascular smooth muscle [5].


Fig. 5.2
Organisation of the capillary neurovascular unit. (a) Rings of smooth muscle encircle arterioles, while pericytes send processes along and around capillaries, without fully covering them. (b) Pericytes are located outside the endothelial cells and are separated from them and the parenchyma by a layer of basal lamina. In the parenchyma, astrocyte end-feet and neuronal terminals are closely associated with the capillary (From [5] with permission)
The BBB differs radically from the blood–tissue barriers found elsewhere. Its ECs, which form the primary barrier, are surrounded by complex tight junctions that prevent the passage of all charged solutes. The formation and maintenance of these is mediated by the release of factors from pericytes and the astrocytic end-feet as shown by mutant mice which are deficient in pericytes [6, 7]. Experiments in which human ECs were co-cultured with pericytes and astrocytes [8] demonstrated that the formation of connections between ECs require the presence of both astrocytes and pericytes. Cerebral ECs also possess very few pinocytic vesicles. Thus, the BBB isolates its vascular smooth muscle, and the brain parenchyma, from the effects of circulating humoral stimuli and ions such as catecholamines and protons [9]. Only small, uncharged lipid-soluble molecules such as O2, CO2 and glucose, and those transported across the membrane by specific carrier systems, pass through the endothelium with any rapidity [10]. In addition, high levels of monoamine oxidase within endothelial cells (ECs) degrade catecholamines, forming an additional ‘enzymatic’ BBB. As a result, CBF is almost completely unaffected by the circulating humoral stimuli that so powerfully influence other vascular beds. In essence the cerebral circulation is ‘selfish’ in that, unlike, for example, the renal and splanchnic circulations, it does not participate in the redistribution of blood flow during emergencies such as haemorrhage and exercise. This very different behaviour of cerebral blood vessels may be related to the fact that the smooth muscle cells and pericytes of the cerebral circulation (and thymus) are derived from the neural crest rather that the mesothelium as in all other tissues [11].
5.2.1 Other Distinguishing Physiological Responses of Cerebral Vessels
Blood flow through cerebral vessels, like that in other tissues, e.g. renal and splanchnic circulations, is increased by raising the partial pressure of carbon dioxide (PCO2), by lowering the partial pressure of oxygen (PO2) and by lowering pH. The mechanisms responsible for these responses are similar to those seen in other tissues, but generally cerebral vessels are more responsive than others to raised PCO2 and lowered PO2. Another widespread link between metabolism and vascular resistance in excitable tissues is the local concentration of extracellular potassium that rises each time an action potential is repolarised (a greater intensity of action potential discharge giving rise to a proportional increase in extracellular potassium). However, there is one key link between metabolic rate and vascular diameter that is used exclusively by the brain and this is the local concentration of the excitatory neurotransmitter glutamate. It appears that evolution has made use of any signal that provides a good index of metabolic rate and that while raised PCO2 [and the protons (H+) formed when it reacts with water] and lowered PO2 are available to all, the brain also makes use of glutamate, a signal peculiar to itself.
The responses of cerebral vessels to the innervation of their vascular smooth muscle (VSM) also differ from those in other vascular beds. For example, within the normal range of arterial blood pressures (60–140 mmHg), sympathetic activation of an intensity that might close down blood flow to the kidney or severely reduce flow to the skin or skeletal muscle has no effect on cerebral blood flow. It does, however, extend the range of autoregulation when ABP is high, protecting the cerebral microcirculation from excessive pressures and flows. Conversely, abolishing sympathetic discharge by cervical sympathectomy or by alpha-receptor blockade extends the range of autoregulation at low levels of ABP (Fig. 5.3).


Fig. 5.3
Electrically stimulating the sympathetic innervation of the cerebral vasculature raises the upper limit of autoregulation, and interfering with it, either by pharmacological blockade or by cutting nerves, reduces the lower limit, but in the normal range of pressures neither stimulation nor blockade affects cerebral blood flow [12]
5.3 Autoregulation
Most organs, such as the kidney, heart and skeletal muscle, match local blood flow to local metabolism. This autoregulation of blood flow reflects the intrinsic ability of an organ’s vessels to determine its own blood flow without any contribution from the autonomic nervous system. It is most simply demonstrated by removing an organ from the body, to isolate it from the influence of autonomic nerves, and perfusing it from an arterial reservoir that can be raised or lowered. Flow through a lifeless system of rigid vessels treated in this way would increase linearly, and in a lifeless system of compliant vessels, such as thin, rubber tubes or dead blood vessels, the line would curve upwards as the rising arterial pressure distends them, reducing their resistance (Fig. 5.4).


Fig. 5.4
Pressure-flow relations through lifeless tubes. Rigid tubes have linear relations in which a doubling of radius increases flow by 16-fold (Poiseuille’s r 4 relation) and they pass through the origin. In distensible tubes, the relation is upwardly bending and tangents to the curve pass through the x-axis. A tangent can only pass through the y-axis if the vessel is alive and showing some degree of autoregulation
In both these cases, all tangents to the pressure–flow relation pass through the origin or through the x-axis. It is only when the vessels are alive, and actively constricting in response to rising arterial pressure, that a tangent can pass through the y-axis, as it does in Fig. 5.4. An experiment such as this is a straightforward way to demonstrate that the vessels of most tissues possess the intrinsic ability to hold blood flow at an appropriate level and has come to define autoregulation. However, the constriction of resistance vessels, when an arterial reservoir is raised, is due to two distinct processes, each of which elicits its own constricting effect. The first is the washout of vasodilating by-products of metabolism and the second is mechanical stretch of vessel walls. These processes are referred to, respectively, as metabolic and myogenic influences. More revealing, but harder to perform, experiments along these lines would look at metabolic and myogenic influences separately by (a) altering the metabolism of an isolated organ, at constant perfusion pressure, by stimulating its neural input or (b) raising transmural pressure of the vessels, at constant metabolism, by raising both the arterial and venous reservoirs by the same amount. Although one cannot denervate a brain as one can a kidney or a heart, similar autoregulation appears to occur in the cerebral circulation.
Autoregulation of cerebral vessels was first observed in pial vessels viewed through a cranial window [13] by Fog in 1937 [14], and this was later quantified by the Kety-Schmidt [15] method of measuring cerebral blood flow using low concentrations of inspired nitrous oxide. More recently the use of transcranial Doppler ultrasound to measure blood flow velocities non-invasively and continuously has revealed the dynamics of the response, demonstrating that flow can recover within 5–10 s of the onset of a hypotensive challenge [16].
An obvious question now is ‘which vessels contribute most to the change in resistance?’ In most tissues, measurements of the pressure drops across various parts of a vascular bed show that the greatest, and most variable, resistance comes from the small arterioles of about 50–100 μm diameter. However, Kontos et al. [17], who observed changes in diameter directly by looking at those vessels they could see through a microscope, found that the larger pial arteries of the cat (those with a diameter of 200 μm) responded most to changes of perfusion pressure within the physiological range. The smaller, precapillary pial arterioles (diameter < 100 μm) dilated only when ABP fell below 90 mmHg, and below 70 mmHg they were more responsive than the larger ones. When ABP was taken to very high levels (>170–200 mmHg), the larger vessels remained constricted but the smaller arterioles failed to sustain their constriction and dilated, accounting for the upward curve on the right hand side of Fig. 5.1. Kontos estimated that extracranial vessels account for 17% of total cerebral vascular resistance, while the surface pial arteries and precapillary arterioles account for 26% and 32%, respectively. This relative importance of larger vessels contrasts with the situation in most vascular beds where smaller arterioles are responsible for almost all resistance changes. However, in these experiments the blood vessels that are buried in the brain tissue were not observed. Indeed, recent work [1, 5, 18] strongly suggests that cerebral capillaries make an important contribution to cerebral vascular resistance and that capillary diameter is controlled by contractile pericytes as described in Sect. 5.4.4.
5.3.1 The Myogenic Response
VSM cells contract when they are stretched by a rise in transmural pressure. This intrinsic response, first described by Bayliss in 1902 [19], usually returns vessel diameter to its control value, but it can, especially in small arterioles, be so pronounced that the final diameter of a vessel is smaller than that before pressure was increased. Figure 5.5a shows an experimental setup that can be used to demonstrate this and Fig. 5.5b represents results obtained. It is as if the sensor is in series with the muscle cells, encoding tension rather than circumference where, according to the LaPlace relation, T ∝ P× radius. So if pressure (P) is raised and held high then tension (T) will not return to its original value until the vessel’s radius is smaller than it was before the test. It is thought that this myogenic response provides a background basal tone against which other vasoactive influences play. It contributes to the autoregulation of blood flow by helping to couple the behaviour of larger feed vessels to that of small ones embedded in active tissue (see Sect. 5.5), but its main role is probably in limiting the changes of capillary pressure, and the resulting fluid shifts between blood and interstitium, which occur during changes of posture. It is therefore concerned with protection against cerebral oedema.


Fig. 5.5
(a) Apparatus for recording the diameter of a small blood vessel as transmural pressure is changed. (b) Changes of diameter observed in two sizes of vessel after a step change of transmural pressure from 20 to 120 mmHg. After an initial distension in response to the rise in pressure, both vessels constrict. The larger (200 μm) vessel returns to its initial diameter but the smaller (80 μm) vessel constricts so forcefully that it ends with a diameter that is less than it started with [20]
There are several broad hypotheses concerning the sequence of events that couples changes in intravascular pressure or stretch with alterations in VSM activity or tone. These include stretch-activated sarcolemmal Transient Receptor Potential, Melastatin-type (TRPM) ion channels, which depolarise the VSM by allowing the entry of Na+ ions, and the classical transient receptor potential ion channels TRPC6 which primarily conduct Ca2+ ions [21]. Also involved is the modulation of biochemical signalling pathways within VSM, length-dependent contractile protein function and endothelial-dependent modulation of smooth muscle tone. Increased intravascular pressure stimulates the production of cytochrome P-450 4A which catalyses the formation of 20-hydroxyeicosatetraenoic acid (20-HETE) from arachadonic acid. 20-HETE is a powerful vasoconstrictor which activates protein kinase C which depolarises vascular smooth muscle cells by closing Ca2+-activated K+ channels thereby opening L-type, voltage-gated, Ca2+ channels. The resulting rise in intracellular Ca2+ constricts the vessel [22].
5.3.2 Metabolic Responses
Whereas the pressure-induced myogenic response dominates in medium-sized intrinsic (parenchymal) arterioles (20–30 μm), metabolic control mechanisms exert their primary effect on the smallest arterioles (less than 20 μm) that are surrounded by metabolising tissue. In the cerebral circulation, as in other vascular beds (e.g. coronary, skeletal muscle) local changes in metabolism are tightly coupled to local blood flow. Several substances have been proposed to link perfusion to cerebral metabolism. These include oxygen, and carbon dioxide levels, and extracellular concentrations of protons, potassium ion, adenosine and lactate. However, the brain has its own mediator that provides the vasculature with an index of neuronal activity. This is the local concentration of the excitatory neurotransmitter glutamate.
5.3.2.1 Inspired PCO2 and pH
CBF is greatly increased when inspired PCO2 is raised. This shows up as a steep, linear rise in CBF within a PaCO2 range of 25–60 mmHg which Fencl et al. [23] showed to be a simple function of calculated CSF pH. So, while protons cannot diffuse through the BBB at any significant rate, CO2 does so freely and then reacts with water to form protons (Fig. 5.6). The system behaves as if arterial CO2 is the primary stimulus, but in fact it is the pH at the receptor site that is transduced into a response by VSM.


Fig. 5.6
Experiments suggesting that CO2 acts, not as molecular CO2, but by reacting with water to form protons [23]. When Cerebral Blood Flow (CBF), calculated from the difference between arterial and venous O2 contents, is plotted against calculated CSF pH rather than PaCO2, the three sensitivity lines coincide
The above experiments show that protons are responsible for the powerful effects of CO2 but they do not show whether the critical changes are extracellular or intracellular. The answer to this was provided by Apkon and Boron [24] who used a pH-sensitive fluorescent dye to follow the intracellular acidity of small cerebral blood vessels while they separately altered intracellular and extracellular pH. They found that vessel diameter responded only to changes in extracellular pH – an arrangement that ‘makes sense’ because it is the job of these vessels to ignore their own metabolism and to respond to the metabolic activity of the nerve cells that surround them.
5.3.2.2 Oxygen
Reductions in blood levels of O2 have little effect on CBF until the partial pressure of arterial oxygen (PaO2) falls below 50–60 mmHg (Fig. 5.7). At lower PaO2, the CBF rises markedly and flow is almost doubled when levels reach the very low value of 30 mmHg. The hyperbolic shape of this curve suggests that the response to hypoxia may be an emergency mechanism activated when other stimuli fail.


Fig. 5.7
Cerebral blood flow is virtually insensitive to the reduction of arterial oxygen until
falls to dangerously low levels. The response to carbon dioxide is much more powerful [25]

Several mechanisms have been proposed to account for the vasodilating effect of hypoxia. One is that it may be largely due to the opening of VSM potassium channels (KATP channels) that are held shut at normal ATP levels. This occurs if hypoxia becomes intense enough to slow down the rate of ATP synthesis by electron transport. Evidence for this comes from experiments on rats where tolbutamide reverses relaxation by hypoxia but not that caused by raised CO2. The tolbutamide mimics the effect of raised intracellular ATP levels that would close these channels [26].
Other likely links between hypoxia and vasodilation are lactate (from anaerobic metabolism) and adenosine, both of which relax VSM, the former by lowering extracellular pH (see Sect. 5.3.2.1) while the latter activates vasodilatory A2A adenosine receptors on VSM. The importance of adenosine is emphasised by the observation that blocking adenosine receptors with theophylline can roughly halve cerebral hyperaemia [27]. Another factor that can vasodilate by opening KATP channels is hydrogen sulphide (H2S) which is described in Sect. 5.5.2.
The great sensitivity to inspired CO2 does not, however, mean that CO2 is necessarily the dominant factor that determines CBF. Indeed when natural stimuli, such as the stimulation of sensory nerves, are used, most studies show that CO2 falls and PO2 rises in excited parts of the brain.
5.3.2.3 Potassium
Raised extracellular potassium increases CBF by dilating vessels. The cell membrane potential is stabilised by the presence of inward-rectifier potassium channels which maintain resting membrane potential when they are opened. They also contribute to vasodilation in response to increased neuronal activation because the repolarisation of every action potential involves the release of some potassium into the extracellular medium which are not removed by sodium pumps with sufficient rapidity to prevent the buildup of extracellular potassium. Raised interstitial potassium relaxes VSM in two ways. Firstly, it stimulates the hyperpolarising electrogenic activity of VSM sodium pumps, the rate of which is normally limited by the relatively low resting level of extracellular potassium. Secondly, the inward-rectifier potassium channels of VSM are activated by modest rises in extracellular potassium. They are potassium-activated potassium channels that hyperpolarise the VSM membrane when opened [28] and relax the vessels by closing voltage-gated calcium channels.
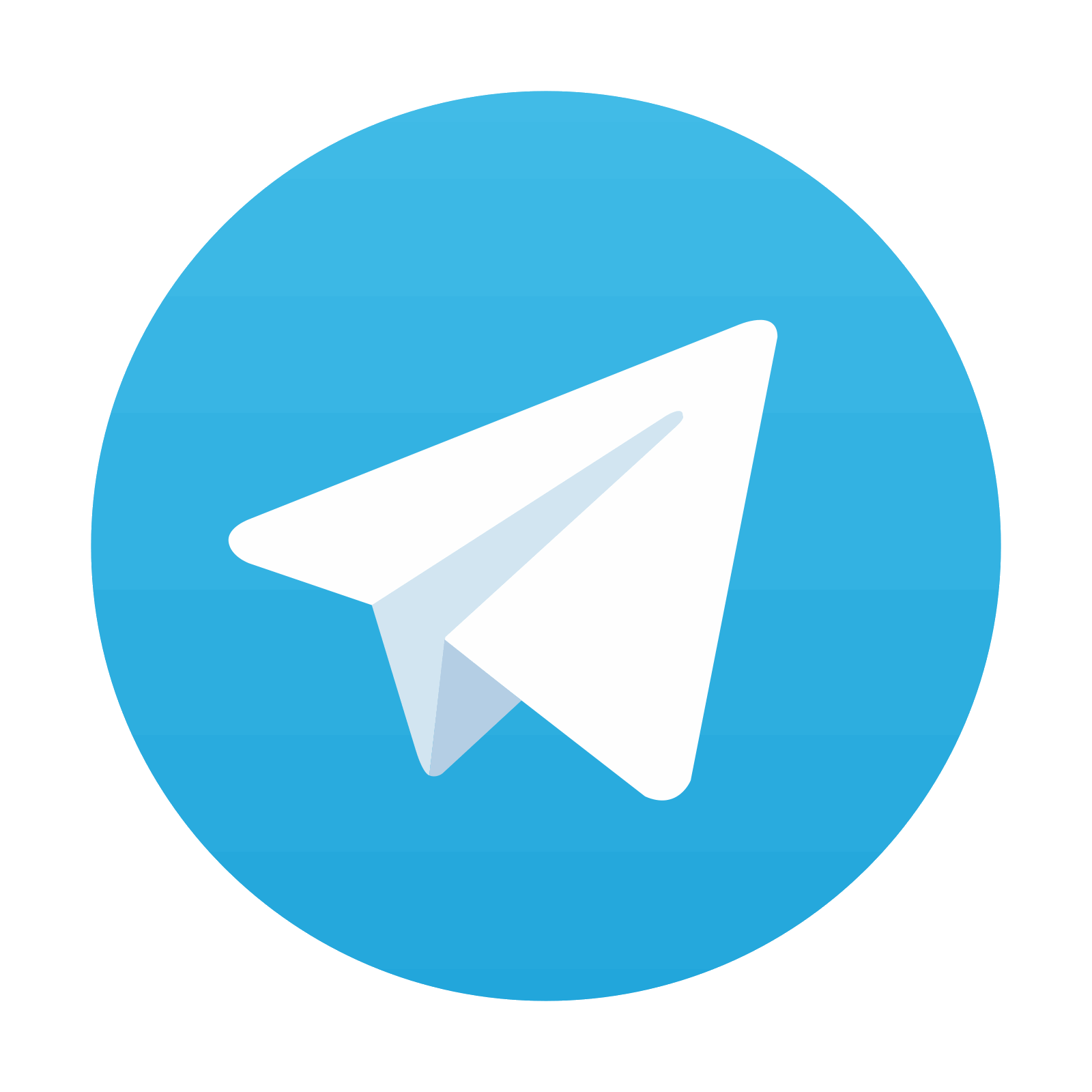
Stay updated, free articles. Join our Telegram channel
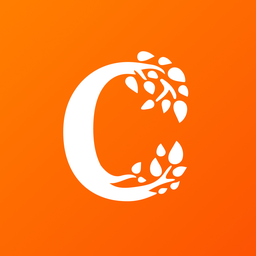
Full access? Get Clinical Tree
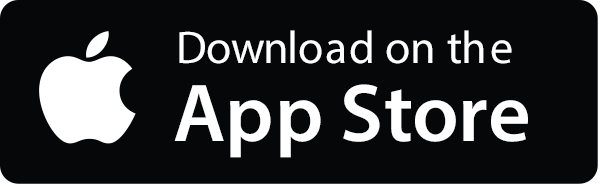
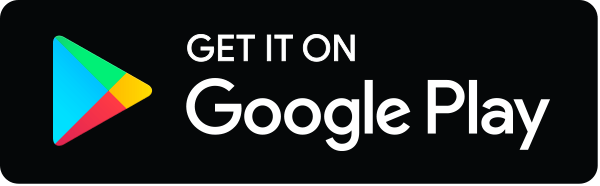
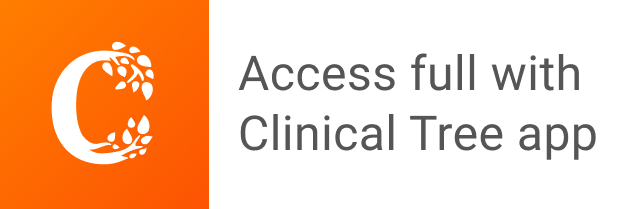