5.2.1 Hematopoieitic Stem Cells
The initial use of UCB for treatment of hematopoietic disorders was based on the ability of HSCs in the UCB to reconstitute the immune system and therefore be a viable substitute for bone marrow. The HSCs are characterized by their expression of CD34, CD45, and CD133, which are hematopoietic lineage-specific antigens (McGuckin et al. 2003; Ali and Al-Mulla 2012). HSCs are not only present in UCB, but also seemed to be in a very early stage of development, as they maintain a very high proliferation rate even after over 20 years of cryopreservation (Broxmeyer et al. 2011). When McGuckin et al. (2003) examined the characteristics of UCB immature cell populations using expression of the lineage-specific antigens CD34, CD38, CD117, CD133, CD164, and Thy-1, compared to bone marrow-derived HSCs, they found that UCB contained more cells expressing an interiorCD34 (int34+) marker while being negative for exterior CD34 (ext34¯). They also determined that the UCB cells lacked exteriorized CD38 while expressing CD133. This pattern of antigen expression (intCD34+/extCD34¯/CD133+/CD38low/−) was more highly expressed in UCB than bone marrow and indicated a more naïve phenotype. In addition to this population of very immature progenitor cells, another more primitive cell subset was also observed. These cells were quiescent stem/progenitor cells and potentially capable of long-term engraftment and ectodermal differentiation ; they expressed intCD34+/extCD34¯/extCD117low (McGuckin et al. 2003)
5.2.2 Mesenchymal Stem Cells
Mesenchymal stem cells (MSCs) are also present in UCB. These cells are capable of differentiating into mesodermal cell lineages, including adipogenic, chondrogenic and osteogeneic cells (Bieback et al. 2004; Ali and Al-Mulla 2012). These cells can be identified by their lack of typical hematopoietic antigens, CD34, CD45, and CD133. Although MSCs are found in low numbers in UCB, they are highly proliferative (Ali and Al-Mulla 2012). The MSC can be isolated from the MNC fraction, forming fibroblastoid cells in culture. MSC identity was confirmed through flow cytometry by expression of CD29, CD44, CD73, and HLA-class I (Bieback et al. 2004), although there was decreased fluorescent intensity of the antigen expression compared to bone marrow-derived MSCs. These MSCs also displayed a slightly different antigen profile, with a lower expression of SH2/CD105 and a higher expression of CD106 . The isolated cells could be induced to differentiate into osteogenic, adipogenic and chondrogenic lineages, confirming their mesenchymal stem/progenitor status.
5.2.3 Endothelial Progenitors
Endothelial progenitor cells (EPCs) are also found in UCB (Ingram et al. 2004). These are highly proliferative cells involved in the vascular genesis, angiogenesis and arteriogenesis. There are no specific antigens that identify all EPCs but most express CD34, CD133 and vascular endothelial growth factor receptor (VEGFR)-2 (Khakoo and Finkel 2005). Unfortunately these cells also share many surface antigens with HSCs (Asahara and Kawamoto 2004), making bioassays the only way to infer their presence. In general, these cells express endothelial surface antigens including CD31, CD105, CD141, CD144, and CD146 as well as von Willebrand Factor (vWF) but do not express CD14 and CD45.
There have been a number of studies that have compared EPCs from UCB, adult peripheral blood (APB) and bone marrow. UCB contained fifteen times more EPCs than adult peripheral blood; these cells formed larger colonies and could be expanded more than 100 passages without significant senescence or decreased telomere length (Ingram et al. 2004). When the CD34+ cells isolated from the UCB MNC fraction were cultured, they initially expressed CD14 and CD45 (Eggermann et al. 2003) and exhibited a monocyte-like phenotype. This expression profile indicates neither progenitor nor endothelial cell lineage. However, after 6 days in vitro, cells began to demonstrate endothelial phenotype, VEGFR-2+, vascular endothelial (VE)-cadherin+, and CD105+, although they remained CD34- and CD133- and CD14+ and CD45+. After about 10 days in culture, the number of cells demonstrating a endothelial-spindle shaped morphology increased .
EPCs have also been found in cryopreserved UCB (Vanneaux et al. 2010). EPCs from fresh and cryopreserved UCB were compared. Daily assessments of potential endothelial colonies were made, looking for cells with an adherent cobblestone-like morphology. The EPCs from the cryopreserved UCB contained significantly fewer endothelial colony forming cells (ECFCs) than in fresh UCB, but they had a shorter average expansion time. Even so, all cells had high CD31, CD133, VEGFR-2, and vWF expression as well as arterial and venous endothelial cell genes. EPCs from both sources were found to be capable of forming tube-like vessels and participate in in vitro wound healing, forming a cell monolayer over scar tissue. Vanneaux et al. also examined the effects of cryopreservation on cell viability and telomere length and found that fresh and cryopreserved UCB cells had no significant differences in viability or in telomere length .
5.2.4 Other Stem and Progenitor Cells
Not only does UCB contain HSCs, MSCs and EPCs, there is evidence that the UCB has additional stem cell populations. Small non-hematopoietic cells have been found in UCB (McGuckin et al. 2005; Zhao et al. 2006); this population of cells expresses markers typically associated with embryonic stem cells , such as the homeobox protein NANOG, octomer-binding transcription factor-4 (OCT4), and sex determining region Y-box two (SOX2) (Ali and Al-Mulla 2012). McGuckin et al. found a population of highly proliferative embryoid-like cells from lineage-restricted immunomagnetic-separated UCB cells (McGuckin et al. 2005). These cells exhibited exponential growth for approximately 5 weeks, after an initial weeklong lag phase. Even secondary non-adherent cells, from the original UCB cell selection, once replated were capable of similarly high growth levels; these cells express some embryonic-specific antigens: OCT4, stage specific embryonic antigens three and four (SSEA-3, SSEA-4 respectively), tumor rejection antigen (TRA)-1-60, and TRA-1-81). During this study, the researchers were able to differentiate this cell type into hepatogenic cells (McGuckin et al. 2005). Another research group also isolated stem-like cells that strongly expressed embryonic markers NANOG, OCT4, SSEA-3, SSEA-4 as well as TRA-1–60 and TRA-1-81 (Zhao et al. 2006); these cells did not express markers of HSCs or MSCs. The cells also weakly expressed human leukocyte antigen (HLA) -ABC, HLA-DQ, CD40, CD80, and CD86. This is a similar immunogenic profile as embryonic stem cells , which have low immunogenicity. These cells were able to differentiate into neuronal-like cells, endothelial cells and functional pancreatic-islet cells.
5.2.5 Immune Cells
UCB also has a full complement of immune cells in addition to the stem cell compartment. There are many naïve T-lymphocytes, B-lymphocytes, and monocytes in the UCB MNC. These important components of UCB likely play a large role in the low incidence of GVHD with UCB transplants (D’Arena et al. 1998). When UCB immune cells were compared to immune cells from adult peripheral blood using flow cytometry, the percentage of B cells and monocytes was similar between the two sources, but T cells were elevated in UCB.
5.2.5.1 Lymphocytes
T and B cells are cells of the adaptive immune system. Common lymphoid progenitor (CLP) cells are cells with the capacity to develop into B-lymphocytes, T-lymphocytes, and natural killer (NK) cells (Haddad et al. 2004). In UCB these CLPs demonstrate considerable potential for lymphocyte generation and express a particular antigen profile: CD45RA+/CD38-/CD7+. Haddad et al. demonstrated that these CLPs from UCB can be further identified by their antigen profile, in order to predict their differentiation lineage. They found that UCB CLPs expressing CD34+/CD45RAhi/CD7+ were more likely to develop into T cells and CD/34+/CD45RAhi/Lin-CD10+ were more likely to differentiate into B-cells when studied in vitro. They observed that to some degree these cells retained potential for alternative lymphocyte lineage differentiation, but tended towards T/NK cell or B cell lineage, respectively. These findings suggest that many of the lymphocytes found in UCB are in an immature state as precursor cells.
Approximately 55–60 % of the UCB MNC fraction is CD3+ T cells (Wedgwood et al. 1997). When the number and phenotype of UCB T cells was compared to T cells from adult blood using flow cytometry, there was both a greater percentage and a greater number of CD3+/CD38+ immature T lymphocytes in UCB (D’Arena et al. 1998). Further, there were more naïve CD4+ and CD8+ T cells, as identified by their co-expression of the CD45RA+ antigen. In adult peripheral blood samples there were more mature CD4+ cells that co-expressed CD45RO. These authors concluded that UCB contained more T cells and that they were an immature phenotype compared to adult peripheral blood.
Harris and colleagues also investigated the phenotype and immunologic profile of the T cells present in UCB (Harris et al. 1992). While they found fewer CD3+ T lymphocytes in UCB compared to adult peripheral blood samples, the majority of UCB-derived T cells expressed CD3/TCR-α/β, indicative of an immature phenotype, as well as the CD45RA naïve phenotype found in approximately 90 % of UCB T cells. They also found another population of CD3-/CD8+ and CD3-/CD7+ T cells in UCB, but not in adult peripheral blood. Both T cells and NK cells lacked the lytic activity of normal adult lymphocytes, but this activity did develop after about 1 week in culture. UCB T lymphocytes also did not respond to IL-2 or HLA stimulation the same way mature T cells did and their own HLA expression was significantly lower in UCB cells versus adult peripheral blood samples. This lack of mature phenotype may contribute to the therapeutic effects demonstrated by the UCB cells.
B lymphocytes are responsible for the recognition of antigens and production of plasma and memory cells assisting in the immune system’s recognition of foreign molecules (Solomon et al. 1999). These plasma cells from activated B cells secrete antibodies, or immunoglobulins (Ig). Fetal B cells are produced from differentiated precursor cells found in the liver and depending on their stage of differentiation, their expression of various surface antigens changes (Abbas et al. 2011). B cell maturity can be attributed to surface antigen expression of CD19, CD20, and Ig expression (Edwards and Cambridge 2006). The first stage of B cell differentiation is marked by the expression of CD19+/CD20-Ig- (pro-B-cell phenotype), continuing differentiation to express CD19+/CD20+/Ig- indicating a pre-B-cell, and becoming an immature B lymphocyte positive for expression of Ig (CD19+/CD20+/Ig+).
Approximately 25–30 % of the UCB MNC is CD20+ B lymphocytes (Wedgwood et al. 1997). In studies that have compared B lymphocytes from UCB and adult peripheral blood, there appear to be similar numbers of B cells as determined by percentage of cells expressing CD19+ and CD20+ antigens (Harris et al. 1992; D’Arena et al. 1998). Interestingly, about half of the B lymphocytes expressed a CD5+ immature phenotype. When immunoglobulin expression was examined, UCB-derived B cells had greater expression of IgM than adult peripheral blood control samples (Wedgwood et al. 1997). Using flow cytometry and PCR analysis the UCB-derived B cells that expressed CD20+ were found not to express IgG. These findings would indicate that the B cells found in UCB are in the pre-B-cell stage. Further, the IgM antibodies bind oxidized polysaccharides, glycoconjugates and phospholipids (Binder and Silverman 2005) on damaged cells, targeting the cells for destruction. In this way, UCB-derived B cells could limit the overall inflammatory response to stroke.
5.2.5.2 Monocytes
Monocytes (CD14+) are cells of the innate immune system. Adult monocytes remove apoptotic cells, scavenge toxins, and differentiate into macrophage and dendritic cells. They produce both pro- and anti- inflammatory cytokines, growth factors such as VEGF, reactive oxygen species (ROS), nitric oxide, prostaglandins, complement and proteolytic enzymes (see references (Geissmann et al. 2008; Auffray et al. 2009) for recent reviews). These cells may express major histocompatibility (MHC) class II antigen and CD11c (Van Voorhis et al. 1983) and therefore can function as antigen presenting cells. During acute inflammation, such as occurs after stroke , the cells are mobilized from bone marrow by interaction of monocyte chemoattractant protein (MCP)-1 with the CCR2 receptor (van Furth and Cohn 1968), and from the spleen in a CCR2-independent manner (Swirski et al. 2009). There appear to be two types of monocytes in the adult – the CD14+ CD16- cells which express CCR2 and produce IL—10 (Ziegler-Heitbrock et al. 1992; Weber et al. 2000; Geissmann et al. 2008) and the CD14+ CD16+ monocytes, which express high levels of CX3CR1 (fractalkine receptor) and secrete tumor necrosis factor (TNF)-α (Belge et al. 2002). These latter cells have been implicated in the acute inflammatory response following myocardial infarction in the mouse while the former infiltrate the ischemic heart later and are associated with tissue remodeling and inhibition of inflammation (Nahrendorf et al. 2007).
Monocytes within UCB are indistinguishable from monocytes harvested from adult peripheral blood in terms of phagocytic activity, ROS production, ability to degrade bacteria, production of many cytokines and expression of CD14 and CD16 antigens (Gille et al. 2009; Filias et al. 2011). Unlike monocytes from adult blood, however, UCB monocytes are not stimulated by hepatocyte growth factor (HGF) (Jiang et al. 2001). Stimulation of UCB monocytes with IL-4 and granulocyte and macrophage colony stimulating factor (GM-CSF) produces fewer dendritic cells compared to stimulation of adult monocytes (Liu et al. 2001). UCB monocytes also produce less IL-1β, TNF-α, integrin α5 subunit, intercellular adhesion molecule (ICAM)-1, and IL-10 than adult monocytes (Le et al. 1997; Brichard et al. 2001; Jiang et al. 2001). While less IL-10 production would suggest that immunosuppressive function of the UCB monocytes could be blunted compared to adult monocytes, UCB MNCs administered 48 h post-MCAO increase protein expression of IL-10 in the spleen and the brain (Vendrame et al. 2005; Vendrame et al. 2006). It is not clear whether the origin of the IL-10 is monocytes within the UCB MNC, other UCB cell populations or endogenous immune cells within the transplanted animals.
5.2.6 Cord Blood-Derived Neural Cell Lines
While the early work with UCB cells focused on expansion of the cells to treat hematologic disease, the more recent focus on developing a treatment for neurologic disease or injury has required different tools. Sanchez-Ramos and associates were the first to report neural gene and protein expression from UCB-derived cells in vitro (Sanchez-Ramos et al. 2001). Using retinoic acid (RA) and nerve growth factor (NGF), they observed cellular development of UCB cells into neural-like cells that expressed glial fibrillary acidic protein (GFAP) and β-tubulin III, expressed by astrocytes and neurons, respectively. They also observed a significant increase in neural gene mRNA expression for pleiotrophin, glypican-4, neural associated growth protein 43 (GAP43), neuronal pentraxin II, and neuronal PAS1 and a significant decrease in hematopoietic genes HLA class II, attractin, macrophage receptor with collagenous structure (MARCO), leukocyte immunoglobulin-like receptor-8 (LIR-8), CD1c, erythropoietin (Epo) and its receptor. Some of the upregulated gene expression coincided with protein expression, as they also detected significant levels of Mushashi-1, β-tubulin III, pleiotrophin, and neuron-specific neural protein (NeuN) proteins. These results were confirmed and extended to describe cells with a neuronal-like morphology in addition to expression of NeuN, neurofilament, microtubule associated protein (MAP)-2 and GFAP (Ha et al. 2001; Ha et al. 2003). Later work suggested that it was CD133+ primitive stem cells present in the UCB that could produce neural cells (Jang et al. 2004). In another study, CD45- cells exposed to fibroblast growth factor (FGF)2 and human epidermal growth factor (hEGF) for 7 days could express β-tubulin III, GFAP, and galactocerebroside (Gal-C) (Bicknese et al. 2002). Similarly, lineage negative cells from UCB exposed to FGF4, SCF and flt3-ligand expressed nestin, neurofilament, A2B5 and Sox2 (Chua et al. 2009). Brain-derived neurotrophic factor (BDNF) was shown to induce neural-like differentiation of UCB MSCs (Lim et al. 2008). Based on these studies, it was a reasonable supposition that the UCB stem cells could produce neural cells and studies to develop UCB derived neural cell lines began.
One of the first attempts to develop such a cell line was reported by researchers in Poland. Bużańska et al first expanded CD34-/CD45- UCB cells in vitro and after sufficient expansion, differentiated the cells with neurobasal media containing RA and BDNF (Buzanska et al. 2002). The resulting cloned cells were positive for nestin, a marker associated with central nervous system (CNS) stem and progenitor cells. From these nestin positive cells, Bużańska et al. were able to further differentiate the UCB-derived cells with RA and BDNF into three neural progenitor cell types, expressing some neuronal and glial markers: β-tubulin III (neuronal marker), GFAP (astrocytic marker), and Gal-C, (oligodendrocytic marker). Four years later, this research group reported that they had established a neural stem cell (NSC) line from the CD34-/CD45- MNCs in UCB (Bużańska et al. 2006; Habich et al. 2006). Culturing the non-adhesive cells with different combinations of epidermal growth factor (EGF), vitamin B27, FGF2, leukemia inhibitory factor (LIF), and antibiotic antimycotic solution (AAS) with high, low or serum free media, researchers were able to establish a NSC lineage. Cells cultured with serum-free media, EGF, LIF, and FGF2 remained in an undifferentiated state as clumps of small round cells without development of an adherent basal layer. Cells cultured in low-serum, EGF, LIF and FGF2 developed an adhesive basal layer and maintained some undifferentiated free-floating cells, which expressed genes related to self-renewal (Wnt, Lif, and TGF-β pathway genes), NSCs (including Notch-2, -3, and-4), and neural commitment (Sox2). Both serum-free and low serum culture conditions yielded slower but continuous rates of expansion up to 20 days in vitro. High serum cultures yielded mostly adherent cell morphologies, and exhibited high initial growth rate, but became senescent. Researchers observed their NSC-line to be clonogenic, at a rate of about 10 %, and demonstrated neural marker expression of nestin, GFAP, and neurofilament heavy (NF-200), when cultured in low-serum media conditions. Once this cell line was developed this research group was able to induce neuronal differentiation ; the best conditions for generation of neurons were low serum and co-culture with rat astrocytes or hippocampal slices (Jurga et al. 2006). They also demonstrated that these cells expressed neurotransmitters and their receptors and were electrically active (Sun et al. 2005); unfortunately, the cells did not have the necessary sodium channels to generate an action potential. It was not clear if these cells were just not mature enough to have developed this ion channel or were not truly functional neurons.
5.3 Effect of Cord Blood Cells After Stroke
In the first study to use UCB cells as a therapeutic for stroke , researchers intravenously (IV) administered UCB cells to rats at two time points, 24 h and 7 days post-Middle cerebral artery occlusion (MCAO) (Chen et al. 2001). They found that their treatment improved rat functional recovery and reduced neurological deficits when administered at 24 h post-MCAO. Although they did not see an infarct volume reduction post-treatment, they were able to identify UCB cells within the stroked brain mostly localized around the site of injury. These results indicated that UCB cells were capable of stimulating functional recovery post stroke, but the mechanisms by which this occurred were unclear.
5.3.1 Which Cord Blood Cells are Most Therapeutic?
The UCB cell preparations reported in the literature have varied across research groups, most likely as a function of the UCB’s heterogeneous nature and the hypothesized mechanism of action. Many researchers choose to employ the MNC fraction or to select a specific cell type, such as MSCs, HSCs or the population of embryonic-like stem cells found in UCB.
Nystedt et al examined the efficacy of UCB CD34+ cells in both transient MCAO using the intraluminal filament method, which produces an infarct encompassing striatum and cortex, and a permanent distal MCAO, in which the infarct is limited to cortex (Nystedt et al. 2006). They transplanted UCB-derived CD34+ cells (5 × 105 cells and 2 × 106, for transient and permanent occlusions, respectively). They failed to observe any change in infarct volume, but the treatments did provide a degree of functional recovery in both MCAO models.
Recently, a study examined the effects of three different fractions of UCB cells administered IV 24 h after stroke (Boltze et al. 2012a). Boltze et al. specifically examined the differences between the use of MNCs, CD34+ cells, and CD34- cells in the treatment of experimental stroke, using both human Jurkat T-cells (hT cells) and non-cell-containing vehicle as controls. Animals administered any type of UCB cells exhibited increased motor recovery compared to those animals that received the hT cells or vehicle control group. CD34+ and CD34- cells improved motor recovery at 7 days post-transplant, whereas, the UCB MNCs took longer to improve motor performance (17 days post-transplant). Although the MNCs did not demonstrate this effect until later in recovery, they exhibited a more pronounced recovery than either the CD34+ and CD34- cell treated groups. MNC treated rats also exhibited decreased CNS tissue loss, significantly more than the CD34+ treatment group, and more than the CD34- treatment group, although not statistically significant. These investigators concluded that UCB MNCs were the best choice for neuroprotection post-stroke, indicating that the combination of the cell types within the MNC enhances the neuroprotective effects of UCB on post-ischemic injury.
We recently published a similar study, but comparing the ability of UCB MNCs to induce stroke recovery to that of the naïve immune cells present in the MNC fraction (Womble et al. 2014). The most profound observation in this study was that almost the entire effect of MNC transplantation was replicated by administering UCB-derived CD14+ monocytes. Similarly, removal of these cells from the MNC fraction reduced recovery and prevented the reduction in infarct size that we normally obtain. These data suggest that the stem cell populations present in the MNC have only a small role in neural repair.
5.3.2 Dose of Cells
An important consideration in the use of UCB cells for cell therapy is the number of cells required to elicit treatment effects. The first study of UCB cells to treat stroke administered 3 × 106 cells IV (Chen et al. 2001). The first study to systematically determine the best dose to use was not performed until 3 years later when we examined the cell dose necessary for IV UCB cell benefits when administered at 24-h post-MCAO (Vendrame et al. 2004). Functional recovery and infarct volume reduction was optimal when UCB cells were given at a dose of 107 cells, compared to lower (105, 106) and higher (between 3 × 107 and 5 × 107) doses. However, when the cells were administered at 48-h post-MCAO, IV cell transplants only required 106 cells (Newcomb et al. 2006). When UCB cell doses of 1 × 106 cells are administered at 48-h post-MCAO they consistently improve function and reduce neural injury (Rowe et al. 2012). Examining tissue for phosphorylated (p)Akt, a protein involved in cell survival pathways, within white matter of stroked brains, investigators found that UCB cell treatment increased ipsilateral pAkt expression at 72 and 96 h post-ischemia. The cells not only reduced infarct volume, especially in the white matter, but also led to an increase in expression of the antioxidant, peroxiredoxin (Prdx)4 and reduced apoptosis as indicated by activated caspase 3 expression post ischemia.
5.3.3 Timing of Administration
One of the major problems with the rtPA treatment for stroke is the very narrow time window it offers: less than 4 h (NINDS rtPA Stroke Study Group 1995). One of the hopes for cell therapy in stroke is that it will allow for a more realistic treatment window to reduce the functional deficits caused by stroke. Various time points have been examined, immediate cell therapy administration and administration within hours or days of stroke onset (Newcomb et al. 2006). Studies suggest that an immediate administration of UCB cells does not demonstrate the same benefits, seen when administered at later time points. Although immediate cell administration has been shown to reduce functional deficits (Borlongan et al. 2004), this immediate administration would provide a smaller therapeutic time window than currently available.
The timing of cell administration affects the efficacy of the treatment (Newcomb et al. 2006). Researchers administered UCB cells IV to rats at one of six time points: 3, 24, 48, and 72 h, 7 days, and at 4 weeks post-ischemia. Previous empirical evidence concluded that 107 UCB cells were necessary for infarct volume reduction when administered within 24 h of stroke onset (Vendrame et al. 2004). However, Newcomb et al found that 106 UCB cells were sufficient to limit the infarct size and prevent cell loss in the penumbra, when given at 48 h post-ischemia. Not only was infarct size smaller, but rats that received cells at 48 h post-MCAO significantly improved motor function, compared to rats with MCAO only or cells administered at other time points. When the cells were delivered at 48 h post stroke, there was a significant reduction in inflammation and apoptosis, which led to reduced infarct volume. This therapeutic time window of 48 h post-ischemic onset would greatly expand the ability to treat and potentially alleviate much of the functional and behavioral damage that currently occurs in stroke victims.
This study was replicated by other investigators. Boltze and colleagues administered UCB MNCs IV at five experimental time windows, post-MCAO: 4, 24, 72, 120 h, and 14 days (Boltze et al. 2012b); the control group was treated with phosphate buffered saline (PBS) vehicle at 24 h post-MCAO. Sensorimotor function and recovery were measured with the modified Neurological Severity Score (mNSS), RotaRod, and BeamWalk assays, every 4 days beginning 1 day post-MCAO and ending 27 days post-MCAO. They found that UCB cells administered within 72 h of ischemic injury reduced stroke-related functional deficits and reduced infarct size even though the IV administered cells were not found engrafted in the cortex.
5.3.4 Route of Administration
Just as there are multiple methods of drug administration for pharmacologic treatment of disease, research for cell therapy explores different methods for cell administration. The chosen route of administration for UCB cells has a few variations, due to an evolution of understanding about the UCB cell mechanisms of action in vivo. There are four main routes of cell administration: intrastriatal (IS), intracerebroventricular (ICV), intravenous (IV), and intrarterial (IA). In both IS and ICV, the cells are administered directly into the CNS, whereas IV and IA routes of administration are peripheral. Although direct, IS delivery is an invasive technique for cell administration as it disturbs the skull, meninges, and parenchyma. After ischemic injury, the necrotic core is a toxic environment for transplanted cells and their survival rate is usually poor (Bühnemann et al. 2006), hence placement near the infarct, but not in it, is critical. IS and IV are the most common methods for UCB cell administration in experimental stroke therapy. Although both methods demonstrate positive effects, such as infarct volume reduction and improved behavioral recovery, IV has become the preferred route of administration for UCB cells in stroke.
The use of IS cell administration has demonstrated success in reducing infarct volume and improving neurobehavioral recovery after an ischemic incident (Koh et al. 2008). The cultured UCB MSC cells were administered by stereotaxic-IS injection, 14 days post-MCAO. The cells were injected ipsilateral to the lesion, in a dose of 6 × 105 UCB MSCs per animal. Animals that received UCB cell treatment showed improved neurological function, based on Neurlogical Deficit Scores, compared to control subjects. The treated animals also displayed reduced infarct volume compared to controls, as measured by magnetic resonance imaging (MRI). Although researchers found some expression of neural markers (NeuN), suggesting neural differentiation of implanted cells, the UCB cells predominantly maintained an undifferentiated state.
We also investigated the optimal route of administration for UCB cell therapy for the treatment of stroke (Willing et al. 2003). Given at 24-h post-MCAO, UCB cells were administered either IV (1 × 106 cells in 10 µl) or IS (250,000 cells in 2.5 µl). Overall, IV delivery produced less cellular debris in the injured striatum and increased functional recovery that was stable over time compared to the IS or stroke control groups, even though there was no evidence of infiltration of the UCB cells into the CNS. We had hypothesized that the cells would infiltrate the infarcted brain since the earlier demonstration that the stroked brain produced chemotaxic signals that attracted UCB MNCs (Chen et al. 2001) that included production of growth regulated oncogene/cytokine-induced neutrophil chemoattractant (GRO/CINC)-1,MCP-1, macrophage inflammatory protein (MIP)-1 (Newman et al. 2005; Jiang et al. 2008). Further, blocking these chemokines reduced UCB homing toward infarcted brain tissue.
A later study addressed the issue of penetration of the transplanted cells into the CNS (Borlongan et al. 2004). The premise was that mannitol, an osmotic that would open the blood brain barrier (BBB), would allow more cell infiltration into the injured brain. While the combination of UCB therapy and mannitol significantly reduced behavioral dysfunction and infarct size as measured 3 days post-MCAO compared to even the group treated with UCB cells alone, they still found few transplanted cells in the brain. Even so, treatment was still able to induce trophic factor expression in the infarcted brain. The benefit of mannitol administration was likely by changing osmotic pressure and minimizing edema as opposed to increasing contact of the UCB cells with neural substrates. It also suggests that the effect of the cells was most likely indirect either through the release of circulating factors or stimulating endogenous cells to produce trophic factors.
5.4 Underlying Mechanism of Action
Initially it was thought that the cells would act as replacement cells for necrotic tissue, especially if administered directly into the brain; it was believed that it was necessary for the cells to localize or be administered directly to the site of injury in order to ameliorate that injury, replacing necrotic cells in the infarct core. However, this view has shifted. Scientists now believe that UCB cells act indirectly through immune modulation and soluble factors (Vendrame et al. 2005). Phenomena such as angiogenesis, neurogenesis, the reduction or cessation of apoptosis and inflammation , as well as increased trophic support have all been associated with UCB cell treatment of stroke.
5.4.1 Cell Replacement
Much of the support for this theoretical mechanism of action stems from the in vitro research of UCB cell transdifferentiation . Although UCB cells are capable of differentiating into cells from endo-, meso-, and ecto-dermal cell lineages under proper cell culture conditions, the evidence is lacking for effective in vivo transdifferentiation and cell replacement. Based on the evidence of UCB cell high proliferation rate and ability to differentiate into multiple cell lineages, we chose to directly implant the UCB cells in the subventricular zone (SVZ) of the rat neonate (Zigova et al. 2002) because it is one of the regions of the adult brain known to maintain neurogenesis throughout life and these new cells typically migrate to the olfactory bulb via the rostral migratory stream. The implanted UCB cells were found mostly in the SVZ but also observed in the corpus callosum and nearby parenchyma; they were not found in the contralateral hemisphere. Survival of the cells was approximately 20 %. Most of the transplanted cells were observed to express GFAP, exhibit astrocyte-like morphology, and were found in the corpus callosum and parenchyma. Expression of neuronal proteins was a rare occurrence, with only about 0.2 % of cells expressing a human antigen and neuron-specific class III β-tubulin (TuJ1). In the adult striatum of NOD/SCID mice, no UCB cells were observed 1 mo after grafting even though 5 % of the UCB cells were present in the striatum at 5 days post- transplant and expressed early neuronal antigens (TuJ1) (Walczak et al. 2007). After MCAO, results are not much different. In the Chen et al. paper, survival of UCB cells within the infarcted hemisphere was approximately 1 % of the cells administered (Chen et al. 2001); few of these cells expressed neural antigens. In subsequent studies, few UCB cells have been found to survive in the brain of stroked rats or mice. Even in studies that have used UCB derived cells that have undergone neural induction prior to transplantation into infarcted cortex, there was a paucity of cells (Kozłowska et al. 2007).
5.4.2 Cord Blood Cells Provide Trophic Support
One of the advantages of using a cell therapy to treat an ischemic stroke is that the living cells are protein factories that are regulated by the microenvironment surrounding them. This cannot be replicated by the administration of a trophic cocktail. As such, the UCB cells can provide potent trophic influences to the injured brain. Studies that have examined the secretory products of the cells report the expression of a myriad of growth factors , cytokines, and chemokines in these cells.
When PC12 cells were exposed to oxygen glucose deprivation (OGD) followed by reoxygenation in the presence or absence of UCB MNCs, mRNA for nerve growth factor (NGF), VEGF, and FGF2 increased in the UCB cells (Arien-Zakay et al. 2009). The CD34+ UCB cells have been shown to express both NGF and the trkA receptor (Bracci-Laudiero et al. 2003). In addition to NGF, mRNA for the other neurotrophins, brain derived neurotrophic factor (BDNF) and neurotropin 4/5 (NT4/5), has also been observed in these cells (Fan et al. 2005). Zwart et al demonstrated that UCB MSCs secreted transforming growth factor (TGF)-β, ciliary neurotrophic factor (CNTF) as well as NT3 and BDNF (Zwart et al. 2009). We confirmed these results and showed that platelet derived growth factor (PDGF)-BB, granulocyte colony stimulating factor (G-CSF) and granulocyte and macrophage colony stimulating factor (GM-CSF) were also secreted constitutively (Chen et al. 2010). In a mouse model of ataxia, UCB cells were shown to express insulin like growth factor (IGF)-1 and VEGF (Zhang et al. 2011). Similarly, UCB MSCs were shown to secrete interleukin (IL)-6, VEGF, hepatocyte growth factor (HGF), and TGF-β (Park et al. 2009). Even the growth factors , and not just the neurotrophins, have been shown to have beneficial effects in rodent models of stroke (Dhandapani and Brann 2003; Schabitz et al. 2003; Zhu et al. 2005; Kawada et al. 2006; Shang et al. 2011; Shen et al. 2012; Wang et al. 2013a).
In addition to trophic/growth factors, we have shown that the UCB cells produce many chemokines and cytokines. The two most prevalent chemokines produced by the UCB cells are IL-8 (CXCL8) and MCP-1 (CCL2) (Newman et al. 2006) and these are produced regardless of the culture conditions the cells were exposed to. The environment of the cells, however, determines whether stromal derived factor (SDF)-1b, also referred to as CXCL12) and leptin are secreted. Hau et al demonstrated that CCL5 (RANTES), CCL3 (MIP-1α), CCL4 (MIP-1β) and CXCL10 (interferon gamma-induced protein 10 or IP-10) all increased in cultures of hypoxic neurons to which UCB cells were added (Hau et al. 2008). Cytokines that the cells produce include IL-1α, IL-6, IL-11, and small amounts of interferon (IFN)-γ and TNF-α (Chen et al. 2010). The cells also produce a number of molecules that are essential for the interaction of the cells with other cells and extracellular matrix, including E-selectin, L-selectin, intercellular adhesion molecule (ICAM), and vascular adhesion molecule (VCAM). While these adhesion molecules are essential for the trafficking of the UCB immune cells from the blood stream into the tissue, they may also be responsible for the ability of the UCB cells to enhance neuritic outgrowth in culture (Chen et al. 2010) and stimulate dendritic growth in other animal models of CNS aging or disease (Shahaduzzaman et al. 2013; Willing et al. In Press).
Any of these trophic factors, chemokines or cytokines may modulate processes as diverse as inflammation, apoptosis, angiogenesis, neurogenesis or the redox system. This is not an exhaustive list, but studies have been performed to examine UCB effects on each of these.
5.4.3 Cord Blood-Neural Cell Interactions
There have also been a number of studies to determine how UCB cells interact with neural cells in the injured brain (see Fig. 5.1). While in vivo studies of UCB cell efficacy examine infarct size, and infer that UCB cells saved neurons when infarct size decreases, there have been fewer studies that have actually measured neuronal survival. In an in vitro model of hypoxia, UCB cells added to the neuronal culture after hypoxia were able to decrease apoptotic cell death to the level observed in normoxic cultures (Hau et al. 2008). In another study, glutamate was applied to either cortical neuronal cultures or co-cultures of cortical neurons and UCB cells. In the co-cultures, neuronal death as a result of glutamate exposure decreased, apoptosis decreased and there was an upregulation of Akt survival pathways in the cultured neurons. We do observe decreased apoptosis in the brains of MCAO rats treated with UCB cells (Newcomb et al. 2006). Even in cultures under normal conditions, the UCB cells can increase neuronal survival and neurite outgrowth (Chen et al. 2010).
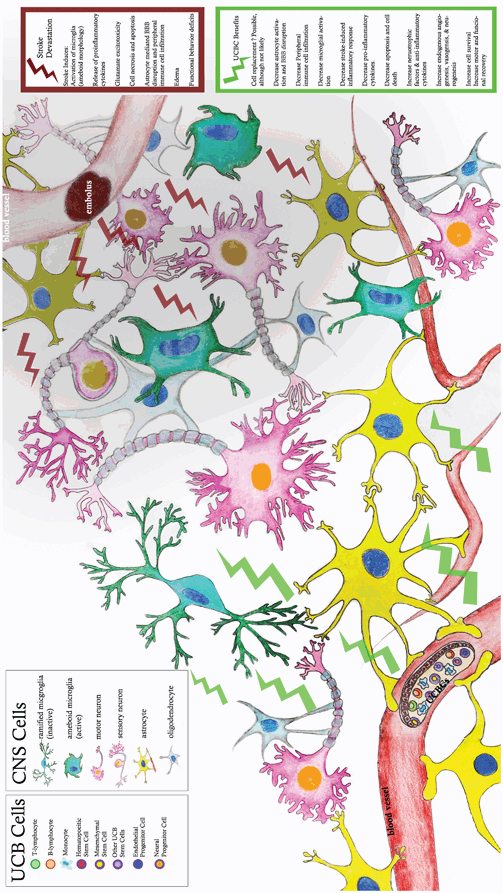
Fig. 5.1
CNS effects of cord blood administration
Hall and fellow researchers found UCB cells have a protective effect on oligodendrocytes in vitro (Hall et al. 2009a). Using the lactic acid dehydrogenase (LDH) assay to determine oligodendrocyte survival, researchers found that UCB cells increased oligodendrocyte survival post-ischemia and also reduced caspase-3 activation, indicating a reduction in apoptosis. Further studies using microarray and quantitative real time polymerase chain reaction (qRT-PCR) assays demonstrated that soluble factors secreted by the UCB cells increased expression of oligodendrocyte repair, proliferation , and survival genes Insig1, Mt3, Prdx4, Stmn2, MOG, and Vcan (Rowe et al. 2010). These UCB effects were mediated by Akt signal transduction pathways, since addition of an Akt inhibitor to the culture medium eliminated the effects of the UCB cells (Rowe et al. 2012); UCB induced peroxiredoxin 4 (Prdx4) expression. Prdx4 expression, an endogenous antioxidant often involved in the reduction of oxidative stress, was prevented when Akt was inhibited. The potential of UCB cells to protect neuroglial cells post-ischemia suggests that UCB cell treatments salvage CNS structure and potentially function as well.
5.4.4 Vascular Repair
In the first study to suggest that the cells could induce vascular repair, IV administration of CD34+ UCB cells 48 h after distal MCAO resulted in the proliferation of endothelial cells and growth of new blood vessels in the ischemic zone around the infarct (Taguchi et al. 2004). Further, if an anti-angiogenic agent (Endostatin) was administered after UCB injection, stroke-induced neurogenesis was inhibited and cortical volume was decreased. More recently, AC133 (CD133)+ UCB cells were examined. There is also some evidence that endothelial progenitor cells express CD34, CD133, and VEGFR-2 (Peichev et al. 2000). Cell culture studies had shown that these cells could be expanded in vitro and produce tube-like structures reminiscent of capillaries (Janic et al. 2010). In a transient MCAO model with AC133+ cells delivered 24 h post-stroke, the cells increased expression of von Willebrand factor around the infarct; however, there was no change in cerebral blood flow (Iskander et al. 2013). In another study, EPCs and smooth muscle progenitors were isolated from CD34+ UCB cells on the basis of clone morphology and expanded in vitro. Twenty four hours after distal MCAO in the mouse, UCB-derived smooth muscle progenitors, EPCs or a combination of both were administered via the tail vein (Nih et al. 2012). All UCB cells increased vascular density in the cortex around the lesion and enhanced proliferation of CD31+ cells. Consistent with the earlier studies, endostatin eliminated the effect.
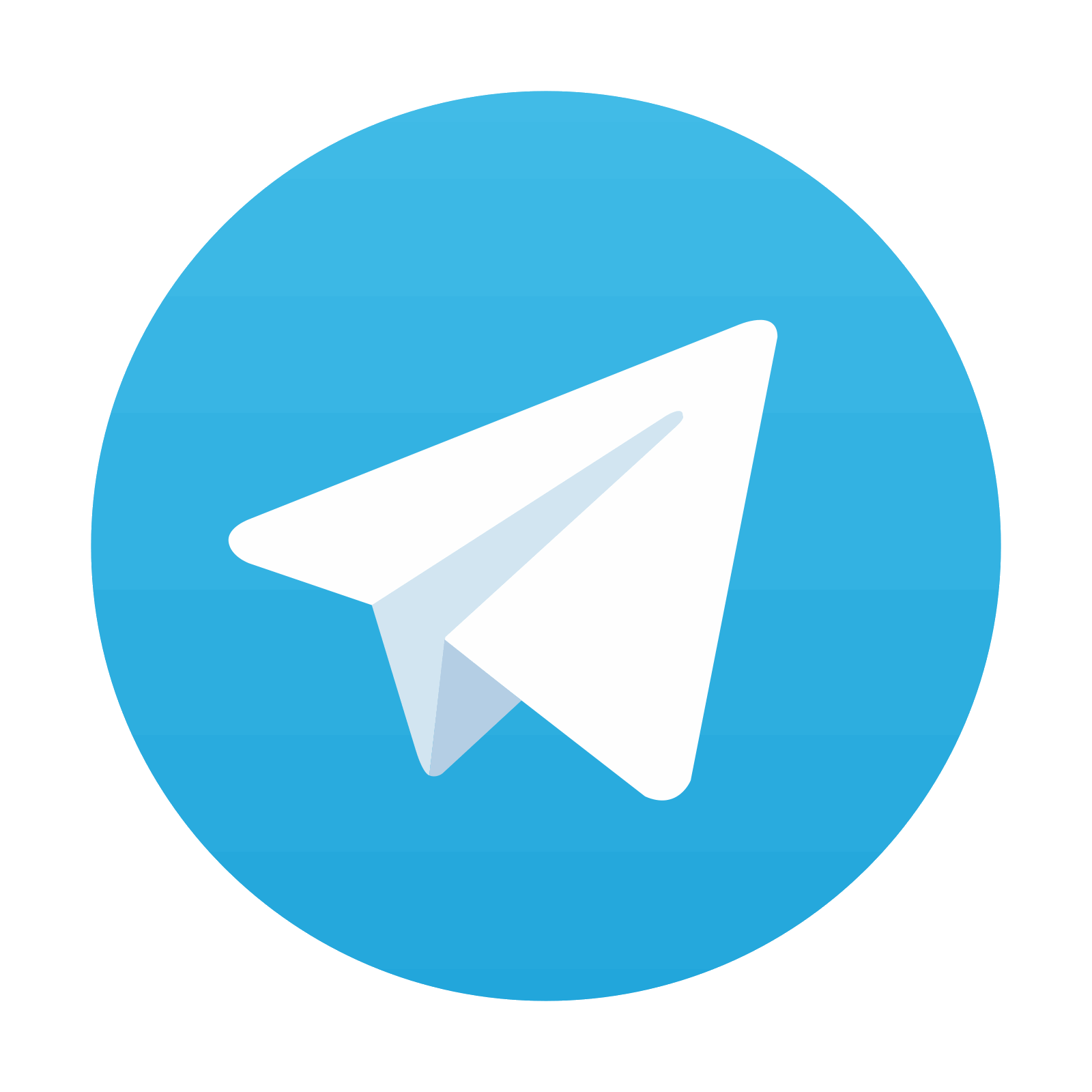
Stay updated, free articles. Join our Telegram channel
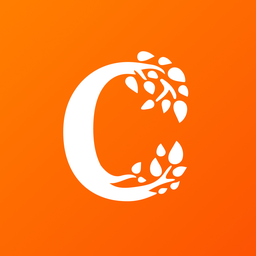
Full access? Get Clinical Tree
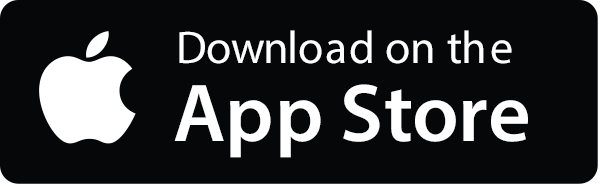
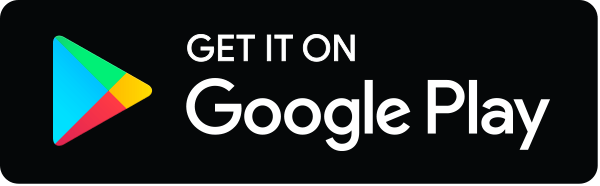