Abbreviations
A
adult
C
cervical
ChABC
chondroitinase ABC
CNS
central nervous system
CSPG
chondroitin sulfate proteoglycans
CST
corticospinal tract
E
embryonic
Htt
huntingtin gene
KLF
Krüppel-like factor
MSE
motor synergy encoder
mTOR
mammalian target of rapamycin
NgR
Nogo-A receptor
NPC
neural progenitor cell
NT-3
neurotrophine-3
P
post-natal
PTEN
tumor suppressor phosphatase and tensin homolog
SCI
spinal cord injury
SOCS3
suppressor of cytokine signaling 3
T
thoracic
YA
young adult
Introduction
The corticospinal tract (CST) is an axonal bundle that starts from cortical layer V pyramid neurons and travels through brain and brainstem to terminate in spinal cord. One major characteristics of CST is that the majority of the CST axons cross the midline at the pyramidal decussation, resulting the right side of brain to control the left side of the body and vice versa. The location of CST is different among different species, from ventral dorsal column in rodent to dorsal lateral funiculus in cat, non-human primate, and human ( ). In addition, the CST termination is different among different species, from dorsal to ventral gray matter.
The main function of the CST is to control primary motor activity of the body, especially the voluntary movements. Disruption of CST along with other tracts after spinal cord injury (SCI) definitely interferes with communication between brain and the spinal cord below the injury, resulting in loss of motor, sensory, and autonomic function. Although studies show that CST sprouts after SCI from uninjured or injured axons, especially after genetically manipulation and therapeutic treatments ( ; ; ; ), true regeneration from transected CST is rarely reported until recently from our lab ( ). Regeneration of CST, including sprouting and regenerative sprouting of CST, is vitally important to restore motor function, especially the voluntary skilled motor function, after SCI.
In this chapter, we will review development of CST and its innervation of spinal cord neurons. In addition, we will review injury-induced CST sprouting, and genetical manipulation and therapeutic treatment to promote CST sprouting and regenerative sprouting. Finally, we will discuss our own work for promotion of CST regeneration by transplantation of caudalized neural progenitor cells (NPCs) after SCI.
CST development
CST outgrowth
The corticospinal tract (CST) is a motor pathway in central nervous system (CNS) starting from the cerebral cortex, traveling through internal capsule and brainstem, most of them crossing the midline at the pyramidal decussation, and ending at the spinal cord ( ). In the popular rodent model of CNS development, CST axons reach brainstem at embryonic day 17 (E17), caudal medulla at E19, and cross the middle line and enter the spinal cord around post-natal day 0 (P0). CST axons continue to extend into entire spinal cord, including cervical cord around P1–2, thoracic around P3, and lumbar around P5–7 ( Fig. 1 ) ( ; ). The development of CST in primate most occurs before birth. For example, CST reaches the entire spinal cord at birth in non-human primate ( ) and reach the entire cervical spinal cord at 24 weeks post-conceptional age in human ( ). The growth of CST is leaded by a small number of “pioneer” axons followed by other axons that eventually populated the CST tract ( ). Following the outgrowth of CST, myelination starts around P10 and finishes in the entire spinal cord around 4 weeks in rodent spinal cord ( Fig. 1 ), indicating readiness for electro impulse transmission ( ).

CST location and termination pattern in spinal cord
CST travels in the spinal cord white matter, which just likes other descending systems in the spinal cord. However, the location where CST travels in the spinal cord varies among species ( ). While the crossed main CST locates in the most ventral portion of dorsal column in rodent, it shifts to dorsal lateral funiculus in cat and primates, including human ( Fig. 2 ). The small proportion of uncrossed lateral and ventral CST are in similar location among different species. In addition, the termination pattern of CST into spinal cord gray matter is different among species. In rodent, the CST terminates most in dorsal and intermediate zone, but not directly into motor neurons in the ventral horn ( Fig. 2 A). However, in primates, both crossed main CST tract and uncrossed CST shift their termination ventrally into intermediate zone and ventral horn ( Fig. 2 C and D), indicating direct cortico-motoneuronal connections ( ). Furthermore, our own work demonstrates additional extensive decussation and bilateral termination of CST in cervical region in monkey, nearly twice as many CST axons decussating in the cervical midline ( ).

Precise control of skilled motor functions by defined CST populations
A recent study identifies spatially defined CST populations with distinct spinal projections that control different musculature groups and function in skilled forelimb motor function in mice ( ). They reveal a sequential activation of topographically organized CST neurons during skilled forelimb performance using in vivo calcium imaging with intersectional approach to specifically label and monitor different regions of CST neurons. Manipulation of region-specific CST neurons by ablation of specific population with diphtheria toxin identifies that caudal forelimb area controls reaching while rostral forelimb area controls grasping. The identification of these spatially defined groups of CST neurons that controls different skilled forelimb movements enables us to understand how CST system precisely works and how to repair it after SCI.
Identification of cellular node for CST control of motor function in mice
Since CST terminate mostly in dorsal and intermediate zone in rodent, it is important to identify those segmental interneurons and propriospinal neurons that relay CST signal into motor neurons. identify a neuronal population term “motor synergy encoder (MSE)” in the mouse spinal cord that may function as relay neurons from CST to motor neurons for voluntary motor movement. The MSE directly receive CST inputs in addition to sensory pathways, and have monosynaptic connection to spinal motor neurons. Molecular study reveals three candidate genes, Tfap2b , Satb1 , and Satb2 , that specifically identify MSE. Identification of MSE could direct neural stem cell study to generate these MSE progenitor cells as graft to attract CST regeneration to mimic their relays for CST input into spinal cord motor neurons below SCI.
Spinal cord injury induced spontaneous sprouting of CST
Classical work
Spontaneous sprouting of spared axons may innervate denervated targets and has been regarded as an underlying factor for functional recovery after SCI. In a classical work to investigate anatomical plasticity of CST, unilaterally lesioned CST tracted at medullary pyramids in young hamster from age 5 to 23 days. They injected vulgaris leucoagglutinin (PHA-L) into intact side to label uninjured CST for 2 weeks. Immunohistochemical study reveals that PHA-L labeled CST sprouts into contralaterally denervated spinal cord side 4 weeks post-injury. CST sprouting is maximal in very young age of 5 days and declines with increased ages. Interestingly, the sprouted CST axons keep the same topographical specificity as normal CST arborization. Their results suggest that sprouting occurs in response to local signals which govern the specificity of denervation and induce sprouting similar to those normal development of corticospinal connections.
Dorsal injury: Ventral CST sprouting
Collateral sprouting after CNS injuries has been proposed as a key mechanism for spontaneous functional recovery. Several studies examined the mechanisms underlying recovery after lesions of motor systems in rodents, including rats and mice. After complete lesions of the main dorsal CST that comprises more than 95% of all CST axons at C3, the uninjured ventral CST spontaneously sprouts into medial motoneuron pools within cervical spinal cord, which correlates with functional recovery ( ). When both dorsal and ventral CST axons are lesioned to remove almost all CST axons, functional recovery abolishes. These results indicate that extensive spontaneous structural plasticity of CST is a mechanism underneath the motor functional recovery after SCI.
Dorsal column injury: Dorsolateral CST spouting
The dorsolateral CST is dispersed throughout the dorsolateral columns. The pathways underlying dorsolateral CST spouting remain poorly understood. By performing optogenetic mapping of motor cortex after a mid-cervical dorsal column SCI that interrupts most corticospinal transmission in mice, demonstrate that the motor cortex can reestablish output to the limbs longitudinally. Importantly, they dissociated for the first time the role of spared dorsolaterally projecting corticospinal neurons in mediating spontaneous recovery after SCI. They found that silencing of dorsolateral corticospinal neurons projecting caudal to the injury site by inhibitory DREADD (designer receptor exclusively activated by designer drug) was sufficient to result in the reappearance of deficits on the horizontal ladder task early after injury ( ). Their results demonstrate that spared dorsolateral CST plays an important role for spontaneous recovery of skilled locomotion in adult mice despite the limited reconstitution of corticospinal density after injury.
Unilateral hemisection: CST cross into denervated side in monkeys
Unilateral hemisection is an important SCI model to assess extensive spontaneous regeneration of adult CST axons that contribute to limited recovery after SCI. This model is especially valid in non-human primates since the main crossed CST locates in dorsolateral funiculus in each spinal cord hemisphere ( Fig. 2 C). To investigate mechanisms underlying spontaneous recovery after incomplete SCI, performed unilateral C7 spinal cord hemisections to adult rhesus monkeys and analyzed behavioral, electrophysiological and anatomical outcomes. They found enhanced spontaneous plasticity of CST axons that cross the midline from intact CST and into denervated injury site below hemisection site with 60% reconstitution of pre-lesion axon density. This extensive CST reconstitution is associated with injured forelimb functional recovery, including coordinated muscle recruitment, hand function and locomotion. This study demonstrates a very extensive CST sprouting to reconstitute injured spinal cord, which highlights the importance of primate model of SCI.
To further dissect the mechanism underlying association of CST sprouting and the forelimb functional recovery, performed C7–8 unilateral SCI in adult macaque and assessed extent of spontaneous recovery of manual dexterity using a reaching/grasping task. They found gradual recovery of the impaired dexterous manual movements. CST from uninjured hemisphere sprouts into the denervated site below injury. However, they found changes of the laminar distribution of CST axons. The sprouting CST axons preferentially innervate into spinal cord lamia IX containing the spinal motor neuron pool, indicating direct innervation of the motor neurons. On the other hand, sprouting CST axons rarely innervate the dorsal laminae. This study indicates that the reorganization of CST axons below the SCI site is an important mechanism associated with recovery of forelimb movements ( ).
Rewiring hindlimb CST
Large thoracic spinal cord injuries in adult rats result in disruption of hindlimb function. To assess whether the hindlimb CST can rewire after thoracic spinal cord injury, demonstrate that transected hindlimb CST axons sprout into the cervical gray matter to contact short and long propriospinal neurons (PSNs). Interestingly, long PSNs naturally innervate lumbar motor neurons, which forms a new intraspinal circuit relay that transmits the hindlimb CST input to its original lumbar spinal targets. In a follow-up study, the same group found additional CST plasticity ( ). They found that injured hindlimb CST sprouts into cervical spinal cord after a thoracic SCI, which forms a new forelimb CST projection in the rostral part of the original hindlimb cortex. They confirm this expansion of forelimb CST in sacrifice of injured hindlimb CST by voltage-sensitive dye (VSD) imaging and blood-oxygen-level-dependent functional magnetic resonance imaging (BOLD fMRI). These studies indicate the existence of great CST plasticity and rewiring after SCI.
Promotion of CST regenerative sprouting
Promotion of CST regenerative sprouting by NT-3
Besides above reports about spontaneous sprouting of CST axons after SCI, many researchers attempt to promote further CST sprouting and regenerative sprouting that could support functional recovery using various treatments. In an early prototype study to search whether neurotrophins can enhance SCT sprouting, found that neurotrophine-3 (NT-3) can enhance CST sprouting first in intact post-natal rats (P2–5) just after CST extends into thoracic cord and next in young adult rats (4–7 weeks) that received low thoracic dorsal hemisection injury. Notably, the main CST is completely transected after injury, and there is no regeneration from cut axon tips into lesion site that is an empty cavity. However, CST axons sprout into ventral cord above injury site and continue to grow along the intact ventral portion of cord to reach caudal cord below injury. Combination of NT-3 single injection with an antibody that can block inhibition greatly enhance long distance of CST growth ( ).
While delivered NT-3 for a single protein injection into spinal cord above injury site, which lasts short period of time, used an ex vivo gene therapy approach to deliver NT-3 by grafting of genetically modified fibroblasts expressing NT-3 into the same SCI model, low-thoracic dorsal hemisection, for continuous local supply of NT-3 in the rat SCI site. Immunohistochemistry demonstrates significant growth of CST axons at and caudal to the injury site comparing to control rats 3 months post-graft. In addition, a significant partial functional recovery on grid walking is reported, which is associated with CST regenerative sprouting. Similarly, delivery of NT-3 by this ex vivo gene therapy promotes chronically (3 months) injured CST regenerative sprouting up to 15 mm distal to SCI site ( ). In another study using gene therapy, a long-term NT-3 in vivo delivery to the lumbar enlargement area through retrograde transport of NT-3 by sciatic injection of adenoviral vector expressing NT-3 promotes CST axonal growth from intact CST into injured side where injury is far rostral at medulla level ( ). These studies indicate that NT-3, especially for local and sustained delivery, support CST axonal re-growth after CST injury.
In vivo NT-3 gene delivery may not be easily translated to clinical application. A recent study implants a one-centimeter long biomaterial chitosan scaffold to slowly release NT-3 into a T8 lateral hemisection site in adult rhesus monkey ( ). Antegrade labeled CST axons regenerate into scaffold and reach the caudal end while there is no regeneration of CST in lesion only control monkey. This is a true regeneration since lateral hemisection completely transects the main CST located in dorsal lateral funiculus and the CST axons grow from their cut ends ( ). However, there is no empty chitosan scaffold control and no quantification of proportion of CST axon regenerated. In addition, this study shows motor and sensory functional recovery. These studies indicate that NT-3, especially for local and sustained delivery, support CST axonal re-growth after CST injury.
Promotion of CST regenerative sprouting by overcome of myelin inhibitors
While certain neurotrophins can function as chemoattractant to attract CST axon to grow, overcoming of inhibitory environment in the injured adult spinal cord could promote further growth of CST. One of the major inhibitions is myelin-associated inhibitors, such as a 35- and a 250-kd membrane proteins named NI-35 and NI-250, initially discovered by Martin Schwab’s group ( ). Afterwards they raised a monoclonal antibody, IN-1, against these two inhibitory proteins ( ). CST sprouts into caudal cord through spared ventral tissue after mid-thoracic dorsal hemisection in young rats (2–6 weeks) after administration of IN-1 produced by tumors intracerebrally. Following this seminar study, various studies were conducted to demonstrate growth of CST axons after similar incomplete SCI model in adult rodent and monkey using IN-1 or its related antibodies termed anti-Nogo-A with or without other treatments, although the number of sprouting axons is very modest and most injury models are hemisection that is rare in human ( ; ; ). Nevertheless, clinical trial using Nogo-A antibody intrathecally infused continuously for 1 month for acute and severe SCI patients started as early in 2006 and finished in 2011 with certain safety data ( ). A phase II trial started 8 years later in 2019 and arrowed down to acute cervical SCI.
In addition to various IN-1 or Nogo-A antibody studies, the cloning of Nogo gene opens a new door to study its receptors for inhibitory signal transduction mechanism as well as CST regeneration in Nogo and its receptor deficient mice ( ). Although early studies report variable amount of CST regeneration in incomplete low-thoracic dorsal hemisection in Nogo mutant mice, a later study re-assessed the early findings and found no enhancement of CST regeneration ( ). Similarly, no enhanced CST regeneration in mice that are deficient of Nogo-A receptor (NgR) that is supposed to mediate inhibition of all three myelin-associated proteins, Nogo, myelin-associated glycoprotein, and oligodendrocyte myelin glycoprotein ( ). Inhibition of NgR with their antagonist peptide NEP1–40 also yields controversy results of CST regeneration ( ). Nevertheless, a soluble function-blocking NgR termed NgR1(310)ecto-Fc, or AXER-204 is tested in rodent, monkey and is in clinical trial at present ( ).
Promotion of CST regenerative sprouting by overcome of CSPG
Besides myelin-associated inhibitors, another major inhibitor is extracellular matrix molecules associated with glia scar around SCI site, such as chondroitin sulfate proteoglycans (CSPGs) ( ). Digestion of CSPG by chondroitinase ABC (ChABC) around C4 dorsal column injury site in rats promotes CST regeneration beyond the injury site, which is associated with functional recovery ( ). Later, the same group delivers ChABC specifically into CST neurons by gene therapy using lentiviral vectors, which reduces CST axon die-back and promotes CST sprouting, but only in short range ( ). Following these exciting results in rodents, delivered ChABC below C7 lateral hemisection by multiple intraparenchymal injections in rhesus monkeys and found that ChABC delivery promotes significant amount of spared CST axonal sprouting from intact side into gray matter below the injury site where it controls hand function. Delivery of ChABC significantly improves hand function comparing with vehicle-injected controls using non-linear principal component analysis. This large animal primate study may pave a path for clinical translation of this strategy to promote CST regenerative sprouting after SCI.
Promotion of CST regenerative sprouting by genetic manipulation of certain genes
The extrinsic inhibitory environment after SCI is not the only factor to prevent CST axonal regeneration. The down-regulation of intrinsic regeneration-associated gene program in the adult neurons after SCI is another important factor for the failure of CST regeneration ( ). A seminal study from Zhigang He’s group shows that the tumor suppressor phosphatase and tensin homolog (PTEN) is one these genes that are responsible to down-regulate growth-associated genes, such as mammalian target of rapamycin (mTOR) ( ). Genetic deletion of PTEN in mice reactivates mTOR expression and promotes substantial amount CST axon growth after SCI. Subsequently, the same group finds another cortical suppressor of cytokine signaling 3 (SOCS3) and deletion of SOCS3 can promote CST sprouting from uninjured CST axons to the denervated spinal cord in a unilateral pyramidotomy model ( ). Co-deletion of SOCS3 and PTEN significantly enhances CST axon sprouting, which results in significant recovery of forelimb skilled locomotion. The deletion of PTEN and SOCS3, however, is at development stage, post-natal day 1, in these studies. A later study demonstrates that diminishing effect of PTEN deletion on CST regeneration in a low-thoracic dorsal hemisection model as the age of mice increases ( ).
Besides deletion of PTEN and SOCS3, directly over-expression of developmentally regulated transcription factors, such as Krüppel-like factor (KLF) family and Sox11, in adult CST neurons by gene therapy improves their regenerative ability after incomplete C4–5 dorsal quadrant injury ( ; ). In addition, a recent study finds that a subunit of voltage-gated calcium channels alpha2delta2 involving synapse assembly negatively regulates CST axon growth and regeneration ( ). Pharmacological suppression of alpha2delta2 with gabapentin promotes CST regenerative sprouting from the injury site into the intact side in a C5 lateral hemisection model in mice. The CST reorganization is associated with forelimb motor functional recovery ( ). Notably, the up-regulation of alpha2delta2 parallels the maturation of CST in the development and the start of synaptogenesis. This indicates that synaptic activity may suppress axonal regeneration ( ). If this new mechanism for failure of axonal regeneration is proved to be true, it opens a new door to enhance axon regeneration by suppressing synaptic activity.
Robust CST regeneration into caudalized NPC graft after SCI
Transplantation of non-neural cells or fetal neural tissue
Although various above studies show sprouting and regenerative sprouting of CST axons after SCI, very few shows that transected CST can regenerate into a large clinically relevant injury site. A large injury site usually contains cavities where no axons can grow without a cellular substrate. Thus, various tissues or cell types are transplanted into SCI sites in combination with neurotrophin or other therapeutic interventions. However, transplantation of non-neural cells, such as fibroblasts, bone marrow stromal cells into the spinal cord lesion site, does not elicit any CST regeneration ( ; ; ). Transplantation of fetal (embryonic day 14) spinal cord tissues in a cervical over-hemisection site, however, does elicit very moderate regeneration of CST axons into lesion/transplant sites ( ). Sparse regenerated CST axons are usually restricted to the rostral a few hundred micrometers. Delivery of BDNF and NT-3 increases regeneration of CST axons, but the effect is very moderate ( ). This study indicates that early stage neural cells could serve as permissive cellular substrates for CST regeneration.
Transplantation of caudalized neural progenitor cells
Based on these previous studies, our group recently modified a transplantation protocol and demonstrated the robust regeneration of CST axons into the caudalized neural progenitor cell (NPC) graft ( ) ( Fig. 3 ). We transplanted freshly dissociated NPCs in single cell suspensions rather than embryonic spinal cord tissues, supported by growth factor cocktail embedding in fibrin matrixes. Transplanted NPCs survive, completely fill in the lesion site, and integrate very well with host tissue, resulting seamless and continuous connections from the transplant to the host tissue ( ). The good integration of NPC transplant with host is critical since regeneration of CST requires direct contact of transected axon stumps with graft ( ). In addition, CST regeneration requires caudalized and homotypic NPC grafts since CST fail to regenerate into telencephalic grafts, less into hindbrain grafts, and mostly into spinal cord grafts ( ).
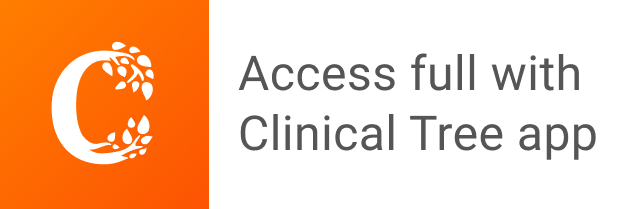