A 64-year-old man is admitted to the hospital with 2 days of worsening abdominal pain and intermittent fevers and underwent a laparoscopic cholecystectomy. On postoperative day one, the patient was hypotensive and oliguric. His blood pressure, heart rate, and urine output did not increase after administration of 3L of Lactated Ringer’s solution. His electrocardiogram showed a sinus rate of 120 beats per minute, his blood pressure was 89/45 mm Hg, and his central venous pressure was 12 mm Hg. Over the course of the morning, his mental status deteriorated, and his work of breathing increased. He was intubated and his lungs were mechanically ventilated. You are called by the resident physician in the postoperative anesthesia care unit and asked to guide further management.
An intensivist assesses a patient’s volume responsiveness to determine if a fluid bolus will increase a patient’s stroke volume and cardiac output. Traditionally, static parameters of cardiac filling pressures, such as the central venous pressure or the pulmonary artery occlusion pressure, have been used as surrogates of a patient’s volume status. Clinicians have also assessed volume status via echocardiographic parameters such as the left ventricular end-diastolic volume or estimated filling pressures. Most of these traditional parameters, however, poorly predict volume responsiveness because Frank-Starling forces, cardiopulmonary interactions, and changes in systolic, diastolic, intra-abdominal, and intrathoracic pressures influence the patient’s response to volume loading.1-4
If a patient lies on the steep portion of the Frank-Starling curve, an increase in preload will increase the stroke volume (volume responsive). On the other hand, if the patient is on the flat portion of the Frank-Starling curve, an increase in preload will not increase stroke volume and indeed may reduce it (non-volume responsive).
Volume assessment aided by dynamic parameters, in contrast to static measurements, recognizes the characteristic respirophasic changes that occur as a patient’s intrathoracic pressure changes with positive pressure ventilation (Figures 34-1 and 34-2). These changes are more dramatic in the hypovolemic patient and can be recognized via the arterial pressure waveform. During a positive-pressure breath with mechanical ventilation, right ventricular (RV) stroke volume drops for two main reasons. First, the RV has less preload because of decreased venous return from an increased intrathoracic pressure. Second, there is a concomitant increase in afterload to the RV secondary to the pneumatic compression of the pulmonary capillaries. Simultaneously, during mechanical insufflation, the venous return to the left ventricle (LV) is increased as the pulmonary capillaries are compressed and blood is pushed to the left side of the heart. Therefore, LV stroke volume increases during mechanical inspiration. After two to three cardiac cycles (pulmonary transit time), the LV stroke volume falls as a consequence of the aforementioned reduction in RV stroke volume and cardiac output. The converse is true for the expiratory phase of mechanical ventilation. As a patient becomes increasingly hypovolemic, this relationship becomes exaggerated and is manifested as arterial line waveform variation from inspiration to expiration.
Figure 34-1.
Cardiorespiratory interactions in hypovolemic patient during mechanical ventilation. RV preload decreases because the increased pleural pressures (1) compress the SVC and (2) compress the RA, whereas (3) the RV afterload is increased in the upper lung regions (West zones I and II). (4) In the dependent lung regions (West III), the pulmonary veins are squeezed and the blood pushed into LA. (5) Higher pleural pressures finally decrease LV afterload. LA, left atrium; LV, left ventricle; Palv, alveolar pressure; Ppl, pleural pressure; RA, right atrium; RV, right ventricle; SVC, superior vena cava. (Reproduced with permission from Michard F. Changes in arterial pressure during mechanical ventilation. Anesthesiology. 2005;103(2):419-428.)

Figure 34-2.
Cardiorespiratory interactions in hypervolemic patients during mechanical ventilation. The vena cava and RA are well filled and thus poorly compliant and compressible; therefore, changes in pleural pressure will have no effect. The pulmonary capillary bed is mainly consistent with West zone III areas; thus, the left preload will increase with insufflation (4) and LV afterload is decreased with increasing pleural pressures. LA, left atrium; LV, left ventricle; Palv, alveolar pressure; Ppl, pleural pressure; RA, right atrium; RV, right ventricle. (Reproduced with permission from Michard F. Changes in arterial pressure during mechanical ventilation. Anesthesiology. 2005;103(2):419-428.)

Dynamic parameters such as the arterial pulse pressure variation, systolic blood pressure variation and pulse contour analysis can be assessed via an arterial blood pressure tracing. Stroke volume (SV) changes can be assessed with echocardiography or an esophageal Doppler probe.
To assess respiratory stroke volume changes with echocardiography, the spectral Doppler function in the apical 5 chamber view is used. Once the Doppler signal is obtained, either the VTI or the Vmax of the SV during end-inspiration and end-expiration is measured. The percentage change is calculated using the following formula:
A ΔVmax of 12% or more was predictive of volume responsiveness in patients with septic shock.5
Measuring IVC variation is another dynamic measurement that is noninvasive and easy to perform.6-8
The IVC returns 80% of the venous blood to the right atrium (RA). It briefly passes through the thoracic cavity before joining the inferior aspect of the right atrium. The vena cava is a collapsible vessel and as such its diameter is dependent on the internal distending pressure and the external compressing pressure. The intrathoracic vena cava’s diameter is determined by the right atrial pressure (RAP) minus the pleural pressure (Ppl). During mechanical ventilation, the greater the pleural pressure the more likely the vena cava is to collapse. As the RAP rises, collapse becomes less likely. This relationship can be evaluated by assessing IVC respiratory diameter and respirophasic variation. The higher the change in IVC diameter, the more likely the patient will respond to fluid loading with an increase in SV and CO. Typical cutoff values that differentiate between responders and nonresponders depends on the method used: 12% (using max IVC diameter – minimal IVC diameter/mean diameter)7 or 18% (using Max IVC diameter – min IVC diameter/min IVC diameter)8 IVC respiratory variability during mechanical ventilation.
Respiratory variation of the superior vena cava (SVC) may also predict fluid responsiveness. In a study of 66 septic mechanically ventilated patients, SVC variability of greater than 36% predicted fluid responsive hemodynamics with a 100% sensitivity and a 90% specificity.9 However, this imaging is only readily obtainable with a transesophageal echocardiography (TEE) and therefore may have limited utility. IVC imaging has the advantage of being easily performed even by physicians untrained in echocardiography.10
The patient should be in a position that optimizes ultrasound windows. For evaluation of the IVC, the patient should be in a supine or semirecumbant position. The patient’s legs may be bent to reduce tension on the abdominal wall. To produce a good ultrasound image there must be copious ultrasound gel between the skin surface and the ultrasound probe. This is important because air will limit the transmission of ultrasound waves, worsening image quality.
It is important to orient the probe properly. Each ultrasound probe has an index marker on one side, which at any time corresponds to the side of the screen marker (Figures 34-3 and 34-4) on the ultrasound machine. It is recommended that a consistent orientation be used.
Two probes are commonly used in the ICU. A linear array probe (Figure 34-5A) has a high resolution, with limited penetration (at most 9 cm). The second probe is a phased array probe (Figure 34-5B) with a small footprint that generates high frame rates to analyze moving organs like the heart and is also able to penetrate deeply into the tissue.
Because the IVC is located deep in the abdominal cavity, a phased array probe is used (Figure 34-5A). After the application of ultrasound gel, place the probe to the left of the patient’s midline in the epigastric area along the longitudinal plane and angled slightly cranially. From top to bottom, the image obtained will show a short axis cut through the left liver lobe that has a homogenous texture and is hypoechoic (gray color). Below and directly adjacent to the hepatic parenchyma you will see a longitudinal cut through the IVC lumen, which is anechoic (black). Identification of the IVC is enhanced by visualizing the hepatic veins draining from the liver parenchyma into the IVC just underneath the diaphragm. The IVC should continue cranially through the diaphragm into the right atrium. The diaphragm image is a crescent shaped hyperechoic (very white) line that moves with respiration. This image of the diaphragm is a landmark that separates the intra-abdominal from intrathoracic cavities. If the IVC is not visualized, tilt the probe to the right or left side of the patient until the images comes into view. The IVC should be differentiated from the abdominal aorta, which lies in close proximity. The aorta is usually deeper and to the patient’s left side. Additionally, it has a clear hyperechoic layer of connective tissue separating the posterior aspect of the liver and the anterior wall of the aorta, whereas the IVC is directly adjacent to the liver parenchyma (Figure 34-6). The venous wall of the IVC is thinner than the aortic wall. Pulsations may be visualized in the IVC or aorta.
Figure 34-6.
A. To scan the IVC in the longitudinal/sagittal plane, the probe is placed anteriorly in the epigastric space (1). By tilting the probe medially (2), the aorta comes into view. B. This is the image obtained with the probe held in position 1. Image orientation: top, abdominal wall/epigastric area; bottom, posterior/back. Right (screen marker), cranial; left, caudal. L, liver; IVC, inferior vena cava; HV, hepatic vein; arrows, diaphragm.

Alternatively, the IVC can be visualized from a more lateral position with the ultrasound probe placed at the patient’s right anterior axillary line, directing the ultrasound beam medially (with the index marker pointing to the head in the longitudinal axis) toward the patient’s spine (Figure 34-7). The ultrasound beam will pass through the right hepatic lobe, imaging the IVC adjacent to the liver parenchyma.
Figure 34-7.
A. To scan the IVC in a more frontal plane, move the probe from the anterior (1) to the lateral position (2). In this plane, the inferior vena cava (IVC) and the aorta are lying next to each other. B. Image orientation: top, right lateral chest; bottom, patient midline. Right (screen marker), cranial; left, caudal.

Once the IVC is identified, respirophasic variations are assessed via time-motion mode or M-mode. This mode analyzes real-time images as a function of time, along only one line (M-Mode Cursor), making dynamic events appear on static images. Typically the picture consists of horizontal black (anechoic) or white (hyperechoic) bands. Once the cursor appears on the screen you place it within 2 to 3 cm distal to the diaphragm perpendicular to the vessel wall (Figure 34-8). Also the hepatic vein-IVC junction may not cross the M-mode cursor during respirations, because it would lead to a false-positive test result. The IVC will be shown as a continuous anechoic band that varies in size depending on the degree of collapsibility during the respiratory cycle (Figure 34-9). Observing the ventilator or patient’s chest is necessary to determine inspiration and expiration. After the widest and narrowest point of the IVC diameter is measured using the caliper function, the collapsibility index is calculated as described above.
How to obtain the echocardiographic images and measurements necessary to assess changes in SV will be described in the Echocardiography section of this chapter.
Figure 34-8.
Measuring inferior vena cava (IVC) variability: the M-mode cursor is placed 2 to 3 cm distally from the diaphragm (arrows) and distally to the hepatic vein (V). With respiratory efforts the organs will move, and if the hepatic vein junction comes to lie underneath the cursor line, it will create a false widening of the IVC on the M-mode picture.

Figure 34-9.
Calculation of the inferior vena cava (IVC) collapsibility index: A is during expiration, and B is during inspiration during mechanical ventilation. In this example, the patient is fluid responsive with a collapsability index of 60% (maxIVC diameter – minIVC diameter/meanIVC diameter, 1.78 cm – 0.59 cm/1.19 cm = > 60%).

Assessment of IVC variation is most reliable when patients are intubated and synchronous with mechanical ventilation. If the tidal volume is less than 8 mL/kg or the patient triggers the breath on the ventilator, the test becomes inaccurate.11 The sensitivity and specificities for predicting fluid responsiveness are not as strong in spontaneously ventilating patients.12 Patients have to be in sinus rhythm. In atrial fibrillation the variability of the IVC may be largely related to changes in diastolic filling time and not due to intrathoracic pressure changes. Furthermore, respiratory variations in the IVC have only been studied in patients without increased intra-abdominal pressures. One could safely assume that if the patient has abdominal hypertension or even compartment syndrome, that the compliance of the IVC is limited and subsequent analysis of variation in diameter may not be accurate. Most of these limitations also apply to other methods of fluid responsiveness using respiratory variations of SV.
Visualization of the IVC may be limited in obese patients. Air limits ultrasound penetration so tissue emphysema, bowel loops, recent laparotomy will all affect image quality. Well-aerated lung tissue may also extend into the costophrenic angles and come to lie between the ultrasound probe and the liver and will intermittently obscure the picture.
During spontaneous ventilation the IVC diameter changes are more dependent on the decrease in intrathoracic pressure than on the preload reserves.13 Because the degree of inspiratory effort and thus decrease in pressure varies from breath to breath, the IVC collapsibility is only poorly correlated to preload responsiveness.12 This is true for patients breathing spontaneously without mechanical assistance as well as intubated patients who trigger the ventilator (eg, assist control mode).14
Methods to assess fluid responsiveness in spontaneously breathing patients rely on challenging the heart with a reversible or minimal fluid bolus. One technique that has repeatedly been shown to be useful is the passive leg raise test. This test is performed by elevating a patient’s legs passively by tilting the bed cranially from a semirecumbent position and measuring a dynamic parameter before and after the test.15 When using this method, the patient is challenged with increased preload with the advantage of total reversibility by putting the legs down. Alternatively, the minimal fluid challenge with the rapid infusion of 50 mL crystalloid or 100 mL colloid fluid has been described.16,17 The volume challenge here is not reversible but is considered too small to cause adverse effects. Investigators have used the pulse-pressure variation, the aortic flow variation (Vmax) via esophageal Doppler18 or the VTI (velocity time integral) variation in the left ventricular outflow tract (LVOT) via the Apical 5-chamber view19 to assess changes in SV (ie, fluid responsiveness). However its ultrasound-based methods for SV variation seem to be more accurate than those based on pulse pressure variation.20 Using the increase in mean arterial pressure (MAP) has shown conflicting results and is not recommended.
For the focused transthoracic echocardiographic exam of biventricular systolic function the following four views are most commonly used: parasternal long-axis (PS LAX), parasternal short-axis (PS SAX), the apical four-chamber (A4CH), and the subcostal four-chamber (SC4CH) (see Figure 34-10).21
Figure 34-10.
TTE windows for the assessment of biventricular systolic function. PSLAX, parasternal long axis; PSSAX, parasternal short axis; A4CH, apical four chamber; A5CH, apical five chamber; A2CH, apical two chamber; A3CH, apical three chamber; LVOT, left ventricular outflow tract; AV, aortic valve; LV, left ventricle; LAX, long axis.

The PS LAX view is obtained by positioning the transducer in the left 3rd or 4th intercostal space, along the anterior mid-clavicular line, with the transducer marker directed towards the right shoulder. This view is primarily used to evaluate left and right ventricular size and systolic function, and to obtain quantitative measurements of ventricular size and wall thickness by M-mode.
The PS SAX view is obtained in the same transducer position as the PS LAX, with the transducer rotated 90° clockwise and the marker directed toward the left shoulder. Within this view, multiple planes of the left ventricle (LV) can be imaged depending on the tilt of the transducer. When tilting the probe from superior to inferior, visualization starting with the basal- and mid SAX of the LV, down to the apical segment of the LV is possible. This view is best used for evaluation of LV size and systolic function. It is optimal for describing regional wall motion abnormalities, as all territories of coronary perfusion can be visualized simultaneously.
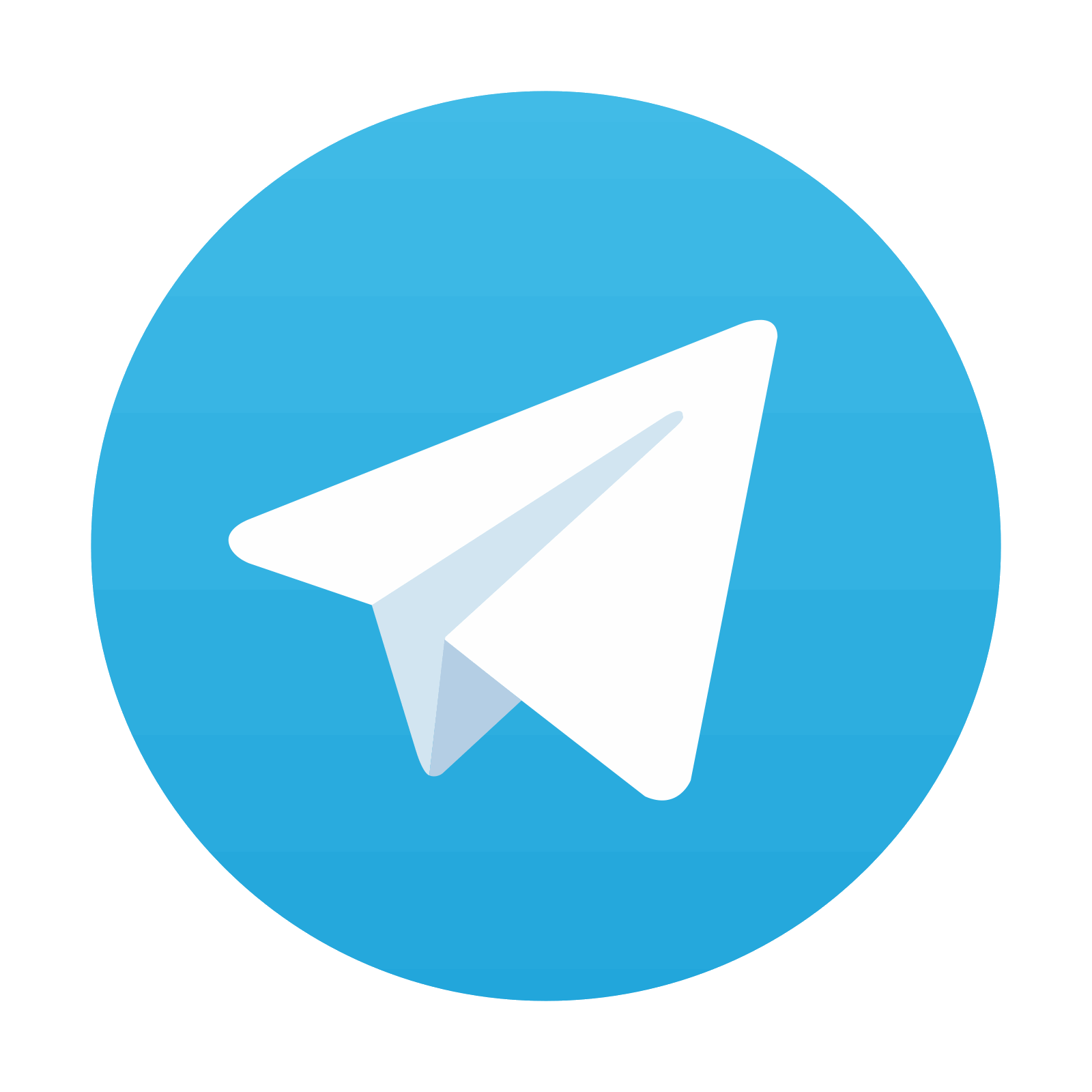
Stay updated, free articles. Join our Telegram channel
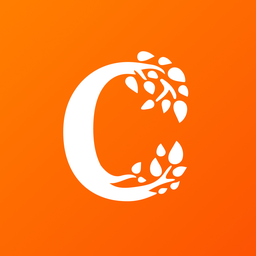
Full access? Get Clinical Tree
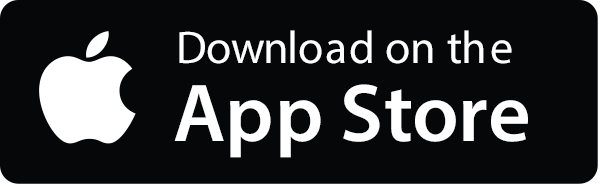
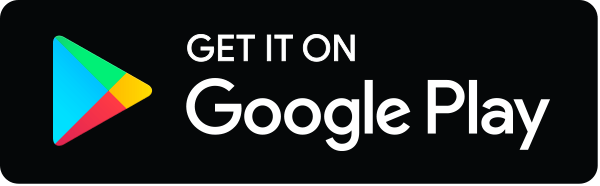