© Springer Science+Business Media Dordrecht 2015
Manuel F. Casanova and Ioan Opris (eds.)Recent Advances on the Modular Organization of the Cortex10.1007/978-94-017-9900-3_1111. Cytoarchitectural Modules: Functional Specialisation and Disruption in Neuropsychiatric Disorders
(1)
Neuropathology Department, University of Oxford, West Wing, Level 1 John Radcliffe Hospital, Headington, Oxford, OX3 9DU, UK
Abstract
Central to the concept of a modular brain is the notion that ‘units’ of function can be identified. Although structural units corresponding to these functions are not a necessary consequence of the model, the presence of modular structures suggests a neural basis. Three levels of structural modular organisation–cortical region, macrocolumn, minicolumn–are considered here, with a focus on the smallest units, the minicolumns, which provide an index of the specialisation and functional integrity of the larger modules. The issue of processing specialisation is discussed with regard to functions that may themselves be considered to be modular: face processing and language. The issue of functional integrity is approached through the association between pathology, minicolumn disruption and functional abnormality in Alzheimer’s disease and schizophrenia. In such disorders there is evidence of pervasive functional disruption across multiple domains which suggests commonalties across modules. In dementia and old age a process of ‘dedifferentiation’ has been observed whereby functions become simplified and less distinct. This appears to be the opposite of ‘emergent modularisation’ that is observed during development. In this context, the degree of modularity of the brain may be considered to change over time, initially increasing and then decreasing across the lifespan. The widespread structural motif of the (mini)column is a simple modular component, common to most brain regions but varying in relation to regional processing biases. Systematic regional variations may develop to support emergent modular function and acquisition of expertise, or may be eroded, reflecting vulnerability to disease and loss of specialisation.
Keywords
MinicolumnMacrocolumnAlzheimer’s diseaseSchizophrenia11.1 Vision and Face Processing
The visual cortex is an archetypal example of modular brain organisation. Its hierarchical, well-characterised structure has been taken as representative of cortical organisation in general, although the clarity of its systematic organisation may, in fact, be rather exceptional. The feedforward-feedback arrangement of visual processing stages from primary to secondary to tertiary and onwards, spans several cytoarchitectonically distinct brain areas. The visual cortex is also famous for its columnar functional organisation constituting modular organisation at a sub-regional scale consisting of patches or ‘macrocolumns’ with a diameter of approximately 500–800 μm. Smaller still are the minicolumnar units with a diameter of approximately 50 μm, which have been measured structurally in both the primary visual cortex and at the far end of the cortical visual stream in the object-specific, face processing cortex. Of these three levels of modular organisation–cortical region, macrocolumn, minicolumn–this chapter considers the evidence that the smallest units, the minicolumns, are building blocks, providing an index of the specialisation and functional integrity of the larger modules. The issue of processing specialisation is considered with regard to functions that may themselves be considered to be modular: face processing and language, by focusing on aspects of these that are asymmetrical between the cerebral hemispheres. The issue of functional integrity is approached by considering the association between pathology, minicolumn disruption and functional abnormality in Alzheimer’s disease and schizophrenia.
Central to the concept of a modular brain is the notion that ‘units’ of function can be identified. Although structural modules corresponding to these functions are not a necessary consequence of the model, the presence of modular structures suggests a potential link and an opportunity to understand the neural basis of these functions. Indeed, past failures to match measures of structure and function may be due to attempts to match incompatible levels of modular organisation (e.g. attempting to match the variation of a small structural unit with characteristics of a function that actually emerge from the interaction of multiple larger brain regions).
In visual cortex, the most commonly investigated modular components are the macro-columns: ocular dominance and orientation preference columns. This columnar organization is dependent on excitatory competition and cell plasticity. Glutamate (NMDAR1) receptor function is required for the development of orientation preference (Ramoa et al. 2001) and manipulation of GABAergic inhibition perturbs the development of columnar architecture (Hensch and Stryker 2004). Markers of dendritic arborization also reflect macrocolumn organization during development (Fenstemaker et al. 2001). For example, SMI32 neurofilament protein is absent at ocular dominance column borders and as a result of monocular stimulus deprivation its distribution is altered. In monocularly deprived dominance columns, MAP2 is reduced, concomitant with a reduction in size of the deprived columns. Myelin staining also varies in distribution according to macro-columnar organization during development. It is more intense in ocular dominance column centers compared to the column borders and becomes darker and wider in columns dominant due to monocular stimulus deprivation.
The macro-columns observed in primary visual cortex do not have clear homologues in many other cortical regions. However, the smaller units, the minicolumns, have been measured throughout cerebral cortex. The horizontal expansion of cortical surface during development (within individual brains), and across evolutionary time (between species), is largely due to the proliferation and spacing of these radial minicolumns of cells (Rakic 1995). These structures persist throughout most cortical areas in the mature brain, where they span the 3–4 mm depth of the cortex with a horizontal width of approximately 50 μm. Column-like radial organization is found for cell bodies and their axonal and dendritic connections. Recent single unit electrophysiological recordings have demonstrated that cells within the same minicolumn share greater similarity of stimulus sensitivity than with cells in neighbouring columns (Opris et al. 2012).
Further down the ventral visual stream, the face processing area in the mid-fusiform region of the temporal cortex (approximately Brodmann area 37 in human brain), is part of the brain network supporting social cognition in humans and other primates. This area specialisation arises from expertise in certain sub-categories of visual object. The high heritability of face processing (Zhu et al. 2010) makes it plausible that there is a detectable neuroanatomical correlate. Although humans and other apes perceive faces holistically (see Taubert and Parr 2010), this process is clearly lateralised in humans—individual facial features are detected in the left hemisphere whereas holistic analysis is biased to the right hemisphere (Rossion et al. 2000). The right hemisphere is dominant for making categorical (face vs non-face) distinctions (Meng et al. 2012). It is reasonable to consider that structural asymmetry in this region may be related to its known asymmetrical function. In humans, cells have become large and less densely packed in the evolution of mid-fusiform cortex compared to the chimpanzee and this is accentuated in the left hemisphere to generate an inter-hemispheric asymmetry. In a study of minicolumn structure narrower minicolumns (and smaller neurons) were, correspondingly, found in the right hemisphere, i.e. the hemisphere that is usually dominant for face perception (Chance et al. 2013).
In the visual domain of face processing it has been proposed that wider minicolumn spacing is associated with detailed feature processing whereas narrow minicolumns may facilitate holistic, configural processing of the type usually associated with face processing (Chance et al. 2013). A mechanistic model is required to explain this. It has been suggested that greater spacing of minicolumns in human association cortex results in less-overlapping dendritic trees and allows more independent minicolumn function (Seldon 1981a, b). Applying this principle, holistic, configural processing for face recognition potentially benefits from the computational overlap generated by narrow minicolumns in the fusiform gyrus. Such a mechanistic interpretation is consistent with the correspondence between the rightward lateralisation of holistic face processing and the narrow minicolumns found in the right hemisphere in humans and is replicated in the structure-function correspondence found in the auditory domain although the processing demands of acoustic processing lead to different hemispheric dominance (described below). The hemispheric processing bias for a given task may depend, therefore, on the degree to which task success emphasizes local or global processing and the hemispheric asymmetry of minicolumnar units in the brain region associated with that functional domain. This concept refines the simple notion that, in terms of cerebral asymmetry, a larger brain area is associated with dominance for a function and offers an alternative, mechanistic explanation associated with ‘processing type’ (Chance et al. 2013). As a result, minicolumn width appears to be dissociated from ‘dominance’, per se, and instead relates to the type of processing: featural or holistic.
11.2 Auditory Cortex and Language
Functional modules are often characterized as domain specific. This specificity depends on a combination of the type of processing that is uniquely associated with the given module and the particular connections restricting the input to the module. In neural structure, the restricted inputs may be seen in the form of specific white matter connections. With regards to processing type, the specificity may also be seen, as suggested in the mechanistic model (above), in the minicolumnar organisation. The minicolumns are structural modules in the cortex that are almost ubiquitous but their systematic variation in width and spacing between brain regions reflects processing specialisation. This variation, thought to be related to the computational independence of units in the local network, confers certain processing characteristics utilized by multiple modules across different domains of processing. An example is seen in the comparison of face-processing with auditory language processing for which similar patterns of structural components confer functional biases with different hemispheric dominance depending on the domain of processing.
Auditory cortex in the superior temporal gyrus (STG) develops a clear columnar cell distribution by the third trimester of fetal life, which is established in early childhood, although axonal maturation continues up to at least 12 years of age (Moore and Guan 2001) and probably later in more associative regions. The planum temporale (PT) in the posterior STG is the most consistently asymmetrical brain area since Geschwind and Levitsky’s original observations (Geschwind and Levitsky 1968). It may be further subdivided into medial, lateral and caudal parts, each associated with different aspects of speech processing (Tremblay et al. 2013). Anterior STG is sensitive to syntactic word category violation in a sentence (Friederici et al. 1993) while the posterior STG supports a left-hemisphere bias for phonological processing (e.g. Robson et al. 2012). Meanwhile, the right hemisphere auditory areas are dominant for music perception in untrained listeners (Ono et al. 2011). Therefore the evidence suggests that lateralised functions (e.g. language) often depend on multiple modular components (e.g. phonology, prosodic intonation etc.) and structural asymmetry (e.g. Sylvian fissure length) depends on structural sub-region components (e.g. anterior, posterior STG and sub-regions of PT).
In the human planum temporale, minicolumn width asymmetry is associated with surface area asymmetry (Chance et al. 2006a). Notably, the asymmetry of minicolumn spacing is absent in the equivalent areas of the brains of other apes (Buxhoeveden et al. 2001). Although the human minicolumn asymmetry is not large (Buxhoeveden et al. 2001; Hutsler 2003), it is estimated to account for a surface area asymmetry of eight to nine percent of the region’s size (Chance et al. 2006a). The asymmetries appear to be amplified in more recently evolved association cortex where the phase of expansion has greater influence on region size and asymmetry (Chance and Crow 2007). Pearlson et al. (1997) have suggested that measurement of surface area is more important than volume and Barta et al. (1997) detected asymmetries by surface area measurements that were not detected by volume measures. The microscopic asymmetry in humans is also detected at the slightly larger scale of inter-connected ‘macrocolumn’ patches in auditory cortex which are more widely spaced in the left than in the right auditory association cortex (Galuske et al. 2000). The cells of a macro-column are selectively interconnected with those of other macro-columns which share a similar stimulus sensitivity.
The wider minicolumn spacing in the left STG is thought to facilitate fine temporal discrimination because minicolumns function as more discrete computational elements, whereas narrow minicolumn spacing in the right STG supports broad spectral processing, due to the minicolumns’ greater computational overlap. In such a scheme, music processing is therefore similar to face processing, based on overlapping, holistic processing. We have found that asymmetry of the minicolumnar organisation of cells in PT reflects axonal interhemispheric connectivity through its connecting region–the isthmus–of the corpus callosum (Chance et al. 2006a). Greater asymmetry is associated with fewer axons, presumably reflecting more independent hemisphere function.
There is not such a tight association between minicolumn spacing and cortical surface area in Heschl’s gyrus (HG) as there is in PT (Chance et al. 2008). Variation in HG size appears to be more dependent on the early established proliferation in number rather than spacing of minicolumns. By contrast, the size and asymmetry of PT is linked more closely to the spacing of its minicolumns. It has been proposed, therefore, that cortical surface expansion in association cortex depends more on the later developmental expansion of minicolumns after their initial proliferation than in primary sensory brain regions (Chance et al. 2008). Regional differences between HG and PT may relate to the hierarchical relationship between them in which PT is the recipient of feed-forward projections from the primary auditory area of HG and plays a role in more integrative, associative processing than HG. The two regions differ in maturation (Guillery 2005; Chance 2006; Toga et al. 2006), dendritic arborization (Elston et al. 1999), asymmetry and neuroplasticity (Arendt 2004). There are also different relationships between minicolumn asymmetry and callosal axon number for HG and PT. An increased number of interhemispheric axons is associated with a more rightward bias for PT and a leftward bias for HG. This may relate to the observation that auditory processing which varies in the temporal domain is processed preferentially by the primary auditory region in the left hemisphere whereas variation in the spectral domain is preferentially processed by the auditory associative areas in the right hemisphere (Jamison et al. 2006). Therefore, it is possible that the domain-sensitive processing bias for each region also depends on callosal interaction.
The normally faster maturing female brain (Kretschmann et al. 1979) is associated with more narrow minicolumns relative to the male brain. It appears that the prolonged development in males contributes to wider minicolumns, larger region size and greater asymmetries in the mature brain compared with that of females (Chance et al. 2008). The corpus callosum goes on developing in size later in females than in males (Cowell et al. 1992; Pujol et al. 1993) continuing through the third and fourth decades of life. Consequently, the sexes differ in their rates of maturation of cortex compared to interhemispheric connectivity. There may be an association between the arrest of axodendritic plasticity seen in the minicolumn data and the peak of callosal maturation (i.e. myelination).
11.3 Schizophrenia: The Disruption of Lateralised Modules and Semantic Memory
The auditory region offers one of the clearest associations between the psychotic symptoms of schizophrenia and brain structure as it is activated during one of the most prominent symptoms of the disorder: auditory hallucinations (Shergill et al. 2000; Ropohl et al. 2004). Reduced cortical grey matter in this area, including the PT, is one of the most replicated structural changes in the disorder (Honea et al. 2005). Minicolumn asymmetry of this region is also altered, in particular in male patients (in whom illness is usually more severe), in such a way that both hemispheres are configured more like the typical right hemisphere (Chance et al. 2008). PT inter-hemispheric connections are implicated by the changed cerebral asymmetry, altered callosal white matter (Diwadkar et al. 2004) and abnormal relationship between callosal axon number and magnopyramidal neuron density in schizophrenia (Simper et al. 2011). We have also reported disturbed associations between cytoarchitectural minicolumn asymmetry and callosal axon distribution in schizophrenia (Chance et al. 2008).
Although neuron density is reported to be unchanged, altered clustering (Beasley et al. 2005) and reduced volume of layer III pyramidal neurons have been found in this area (Sweet et al. 2003). It has been suggested that these findings implicate impaired feed-forward connections in schizophrenia (Sweet et al. 2003) perhaps affecting the interaction between other language areas including prefrontal cortex in Broca’s area. There is a reported asymmetry in the size of magnopyramidal neurons in motor language cortex (Hayes and Lewis 1993) and one may expect a similar asymmetry in Wernicke’s language area (including the PT), which is the sensory equivalent. Indeed, magnopyramidal neurons tend to be larger and denser in the left PT (Simper et al. 2011)
Reduced inhibitory interneuron density has been found in the PT in schizophrenia (Chance et al. 2005). Double bouquet cells are a major class of calbindin containing interneurons. The majority of calbindin-containing cells in the mature brain are double-bouquet cells with vertically oriented dendrites and axon bundles. By exercising inhibitory modulation of pyramidal cells in a columnar arrangement they make possible cohesive vertical inhibition of minicolumns. A bilateral reduction (20 %) in calbindin cell density has been found in patients (Chance et al. 2005). Loss of columnar inhibition may result in reduced minicolumnar segregation and altered cell size appears to reflect altered minicolumn size – calbindin cells are typically larger in subjects with larger minicolumns. High immunoreactivity to calbindin is also found (in layers III and IV) at the borders of macro-columns where it is thought to be associated with synaptic competition on excitatory neurons (Fenstemaker et al. 2001).
In addition to having wider minicolumns, patients do not show the normal minicolumn thinning found in old age. It has been shown that minicolumn thinning occurs during normal ageing without cell loss (Chance et al. 2006b) indicating a loss of neuropil between minicolumns that is likely to be substantially due to dendrite remodelling. Notably, the normal ageing effect is found in association cortex, including the auditory association cortex of PT, but not in the neighbouring primary auditory region of Heschl’s gyrus. The absence of age associated minicolumn thinning in association cortex in schizophrenia suggests a failure of the processes of neuroplastic remodelling. Evidence from neuroimaging, electrophysiology, cytoarchitectural and molecular studies all indicate aberrant neuroplasticity in schizophrenia (Broadbelt et al. 2002; Jones et al. 2002; Stephan et al. 2006). A plausible interpretation is that, as a result of arrested plasticity, the patients retain a larger amount of unmodified neuropil compared to the equivalent elderly controls.
It is worth noting that altered minicolumn asymmetry in the PT contributes to a body of evidence reporting asymmetric changes in schizophrenia. With reference to the face processing regions discussed above, asymmetric volume reduction of fusiform cortex (McDonald et al. 2000) and an asymmetric reduction of minicolumn density in the left fusiform cortex have been reported (Di Rosa et al. 2009). In that study of minicolumns, wider and more asymmetrical minicolumns were found in males compared to females. Findings in the PT have also indicated greater anatomical asymmetry in males (e.g., Chance et al. 2006a). The reduced density of minicolumns in the fusiform cortex in schizophrenia was coincident with a low pyramidal cell density–a logical corollary of a more sparse neuronal distribution–although this was not a statistically significant correlation. These effects are consistent with the notion of anomalous development and the absence of age-associated neuropil restructuring in schizophrenia.
Disrupted minicolumn organisation, reduced inhibition and altered neuroplasticity appear to contribute to the neuropsychological deficits seen in the disorder in both auditory stimulus processing (Rojas et al. 1997) and face processing (Johnston et al. 2005). However, the abnormalities extend beyond these particular domains into multi-modal functions. Indeed, some neuropsychological models of the disorder have struggled to account for the breadth of cognitive deficits identified. Yet, the widespread effect on minicolumn structure suggests a common underlying disruption. As described, minicolumns represent simple modular components with variation related to processing biases. These biases may be scaled up to support emergent modular function in multiple domains. This formulation is consistent with the neuroconstructivist approach (Karmiloff-Smith 2009) whereby domain relevant biases in early development are shaped by experience during subsequent development into emergent ‘modules’. Therefore, minicolumns may not be synonymous with functional modules, but rather, as units in a network they provide a neural correlate for the biases from which functional modules emerge.
In schizophrenia, an example may be seen in the extension of anomalies of auditory processing into the more complex emergent deficits in interpreting linguistic meaning. The loss of left hemisphere ERP mismatch responses to anomalous words at the end of a sentence, based on incongruous word meaning (Spironelli et al. 2008), provides a link between the sensory, phonological abnormalities and semantic category understanding. Patients with schizophrenia have been shown to have altered organisation of the semantic network that encodes basic knowledge about the meanings of words (Paulsen et al. 1996; Rossell et al. 1999). This is supported by several studies indicating that semantic category boundaries are less clear in schizophrenia (Paulsen et al. 1996). The interpretation of this is informed by identifying how the mechanistic description of asymmetrical minicolumn function applies to the domain of semantic processing. In healthy control subjects, a reduced discrimination between primary and secondary word meanings is typically found in the right hemisphere compared to the left (Weisbrod et al. 1998) and this lack of discrimination is consistent with the holistic, overlapping activation in the right hemisphere, as described in the sections above. It also appears to be consistent with the observation that the right hemisphere usually uses more dimensions than the left hemisphere to represent the semantic map in healthy subjects (Taylor et al. (1999)). An alteration of semantic mapping in schizophrenia is identified with less effective mapping of semantic space in low dimensions in patients. Word generation (semantic fluency) tests the integrity of the semantic network and in schizophrenia there is a requirement for more dimensions (Rossell et al. 1999; Paulsen et al. 1996).
If increased number of dimensions is taken to be indicative of more diffuse activation in the right hemisphere network in normal healthy subjects (Taylor et al. 1999), then the hypothesis that schizophrenia patients have unusually diffuse semantic associations in the left hemisphere as well as the right hemisphere (Weisbrod et al. 1998) predicts that patients use more, poorly discriminative dimensions overall. Therefore, alterations in the dimensions of conceptual space, consistent with disruption of lateralised cognitive processing biases, accompany abnormal anatomical structure of the cortex, including altered asymmetrical minicolumn cytoarchitecture in schizophrenia.
Normally a developmental shift occurs in cognition: whereas older children and adults perceive dimensions such as high and tall, or big and bright, to be separable, young children tend to confuse these concepts (Carey 1978). Goldstone and Barsalou (1998) have described the development of reasoning about dimensions: “dimensions that are easily separated by adults, such as the brightness and size of a square, are treated as fused together for children… [they] have difficulty identifying whether two objects differ on their brightness or size even though they can easily see that they differ in some way. Both differentiation and dimensionalization occur throughout one’s lifetime.” Differences in conceptual organisation can be interpreted as differences in the metrics underlying the psychological space (Gardenfors 2000). Gardenfors (2000) has proposed that this is a developmental shift from a Euclidean cognitive metric to the more separable dimensions of the ‘city-block’ metric. The development of more separable dimensions, therefore, is associated with more sophisticated cognitive discriminative ability. Aspects of brain structural maturation and plasticity presumably relate to this process of cognitive maturation. The increase in discrimination associated with separable dimensions is similar to the acquisition of expertise, which is often associated with left hemisphere specialisation for fine-grained difference judgements, e.g. for faces, word meaning and music.
The developmental shift from the Euclidean cognitive metric to the more separable dimensions of the city-block metric proposed by Gardenfors (2000) may be relevant in the neurodevelopmental context of schizophrenia. Although there is a clear genetic component in the aetiology of schizophrenia, onset of illness is not identified until adolescence or early adulthood. It has been proposed that, structurally, this may be linked to the time-course of myelination and altered minicolumn organisation (Chance et al. 2008; Crow et al. 2007). Functionally, it may be linked to the shift in cognitive metric and as dimensionalisation matures, in comparison with typically developing individuals, the anomalies associated with psychosis are exposed, leading to the recognition of ‘onset’ and diagnosis.
11.4 Alzheimer’s Disease: Modular Pathology
It is possible that the modular organization of the cortex from minicolumns, to macrocolumns, to surface regions, determines the pattern of pathological spread in Alzheimer’s disease and, consequently, the pattern of function loss. The progression of pathological changes through anatomically connected regions in AD is consistent with the concept of the disease exploiting the brain’s modular organization at the regional level.
The widely accepted amyloid hypothesis of Alzheimer’s disease is based on the demonstration that mutations in the gene coding for amyloid-β protein precursor (AβPP) cause AD (Goate et al. 1991). Fibrillary amyloid-β, or a precursor form of it, is considered to be toxic to neurons and capable of inducing neurofibrillary tangle (NFT) formation, although the exact mechanism by which amyloid-β causes NFT formation remains unclear. One problem with the amyloid cascade hypothesis is that it does not readily provide an explanation for the regionally selective distribution of NFT observed in AD. NFT accumulation typically appears initially and is most severe in medial temporal cortex. It has been suggested that the vulnerability of the medial temporal lobe, including hippocampus and olfactory areas may be related to the high degree of local synaptic plasticity in these brain regions. There is regional variation in the potential for neuroplasticity in the adult brain. Age-related dendritic growth is most pronounced in limbic cortical areas, while the primary sensory and motor cortex show either dendritic stability or regression (Arendt et al. 1998; Arendt 2003; Arendt 2004). An intermediate degree of dendritic remodeling occurs in association cortex. This variation in dendrites is structurally related to the regional variation in minicolumn spacing as a result of the space-filling properties of the dendritic arbors (Chance 2006).
It has been found that NFT clustering is highly correlated with symptoms: “high numbers of NFTs restricted to small areas are more important in disturbing function than NFTs in a widespread distribution (with the same mean values)” (Nagy et al. 1996). The finding that NFTs are clustered coincidentally across supragranular and infragranular layers provides an important component of the argument that the pathology is distributed to some degree in a modular and columnar fashion. Although plaques also appear to show a degree of clustering (Armstrong 1995) between dendritic clusters (Kosik et al. 1987), the proximity of symptoms to pathology distribution is less striking than it is for tangles.
As an anatomical module the macro-column has a diameter reflecting the tangential spread of afferent projections to the cortex (Seldon 1981b). NFT clustering occurs at a similar scale to that of macrocolumn size. Hiorns et al. (1991) observed that the distribution of the cells of origin of ipsilateral cortico-cortical projections to a given cortical area were clustered in bands of a similar size to macro-columns and a distribution comparable to that of NFTs in AD. Casanova (2003) has anticipated that “the spread of neurofibrillary tangles (NFT) preferentially to corticopetal neurons causes clustering of the neurodegenerative changes which reflect modular structure”. The development of NFT in neurons of layers III and V is consistent with the spatial coincidence of supragranular and infragranular NFT clusters due to shared connectivity of cells in the same macro-column. Consequently NFT clustering may reflect the selective grouping of connections which is fundamental to columnar organization.
Subsequently, the issue of columnar organization and clustering has been pursued with respect to other pathological structures. Disruption of the columnar organization of glial processes has been reported for AD (Colombo et al. 2002). Lewy bodies and Pick bodies (Armstrong et al. 1997; Armstrong et al. 1998) also exhibit clustered distributions. The potency of a link between pathology that clusters and minicolumn abnormality is also borne out by the finding that minicolumns were disrupted even in the absence of an overall loss of neurons, in a group of patients with Lewy body dementia (Buldyrev et al. 2000). In AD, the finding of NFT clustering offers some insight into the roles that plasticity and connectivity play in the spread of pathology.
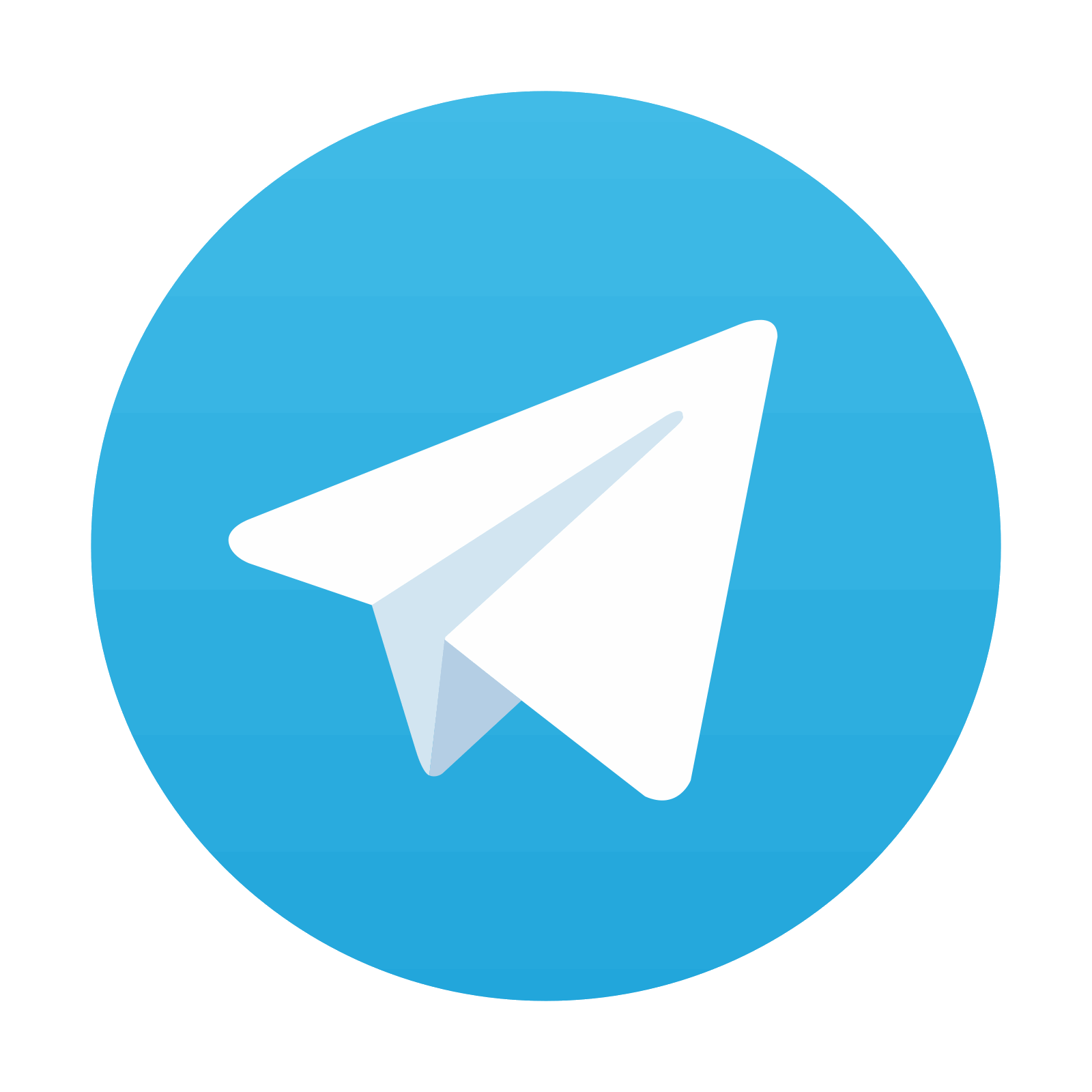
Stay updated, free articles. Join our Telegram channel
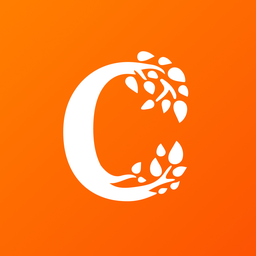
Full access? Get Clinical Tree
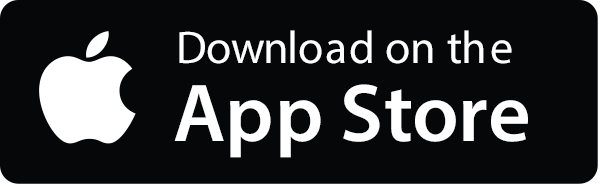
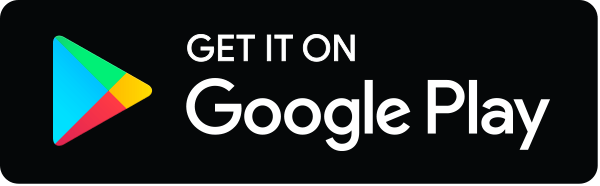