Over the past decade, dendritic cell–based immunotherapy for central nervous system tumors has progressed from preclinical rodent models and safety assessments to phase I/II clinical trials in over 200 patients, which have produced measurable immunologic responses and some prolonged survival rates. Many questions regarding the methods and molecular mechanisms behind this new treatment option, however, remain unanswered. Results from currently ongoing and future studies will help to elucidate which dendritic cell preparations, treatment protocols, and adjuvant therapeutic regimens will optimize the efficacy of dendritic cell vaccination. As clinical studies continue to report results on dendritic cell–mediated immunotherapy, it will be critical to continue refining treatment methods and developing new ways to augment this promising form of glioma treatment.
Dendritic cells (DC) have long been regarded as the most potent antigen-presenting cells within the immune system. Their ability to sample environmental antigens and stimulate T-cell activity in a major histocompatibility complex (MHC)–restricted manner has attracted much attention given the poor antigen-presenting ability and immunogenicity of tumor cells. Although DCs constitute approximately 0.3% of all circulating blood leukocytes, they serve as the sentinels of the immune system and are found nearly ubiquitously throughout the body. In their immature state, DCs are highly specialized antigen samplers capable of surveying their microenvironment through several mechanisms including engulfment, macropinocytosis, and receptor-mediated endocytosis. On encountering an antigen the DC processes it through MHC pathways and directs it to the cell surface to form an MHC-peptide complex ( Fig. 1 ). In line with traditional antigen presentation following uptake from the environment, many antigens are channeled through MHC-class II pathways with resultant MHC-peptide complexes being capable of stimulating CD4+ T cells. In addition, DC possesses the unique ability to “cross-present” acquired antigens. In this process, DC endosomes release captured antigenic material into the cytosol where it is broken down by proteasomes. The degraded peptides are then transported to the endoplasmic reticulum by a transporter-associated protein and bound to MHC-class I molecules for presentation to CD8+ T cells. These distinct mechanisms allow DCs to stimulate T cells in an MHC-class I and II manner, overcoming classical restrictions in antigen processing and presentation and diversifying the resultant immune response.

DCs are capable of handling a vast range of antigenic mediums. The sources of antigen that have been used in DC immunotherapy include exogenous MHC-restricted peptides, acid-eluted tumor peptides, tumor RNA and cDNA, viral vectors, apoptotic tumor cells, tumor cell lysate, and whole tumor cells. Many of these methods have been used with varying degrees of success. A growing sentiment has emerged, however, which argues for the use of a diverse range of antigens that cover both MHC classes rather than constructing specific MHC-matched peptides. The reasoning for this is multifold. First, stimulating T cells with a broad range of antigens reduces the likelihood of an escape phenomenon in which tumor cells lacking the specific antigens of interest avoid immune detection and continue to grow unhindered. Second, it is now well established that the stimulation of both CD4+ and CD8+ T cells is crucial in the activation and maintenance of antitumor immunity. By allowing DCs to present and cross-present antigens on MHC-class II and I molecules, respectively, one avoids having to laboriously engineer peptides for each MHC class. Finally, the methods used to load the spectrum of antigens for a particular tumor obviate the need of characterizing each individual antigen used. Although the use of unfractionated tumor material containing unknown antigens has long raised the concern of inducing autoimmunity, particularly in the form of experimental allergic encephalomyelitis, no reports of this complication have been seen following DC vaccination in humans to date.
DC vaccine is defined as DCs loaded with antigens (eg, those found on glioma), which are administered to patients to induce an antigen-specific T-cell mediated antitumor response. Although immature DC are not functionally ideal for the loading of antigens, they are unable to activate lymphocytes until an inflammatory signal or pathogen induces their maturation. Some groups argue that ex vivo maturation of DCs through CD40L or interferon (IFN)-γ is necessary before vaccine administration to ensure proper antigen presentation and T-cell activation. Others maintain that maturation occurs naturally, and that no prior stimulus is required. In the process of maturation, DCs lose their ability to uptake and process antigens. Moreover, they exchange their immature molecular signature for a mature (CD83+) phenotype, increasing expression of MHC-antigen complexes, lymphocyte costimulatory molecules (eg, CD80/B7-1 and CD86/B7-2), tumor necrosis factor and tumor necrosis factor receptor molecules (eg, CD40), and many chemokines and chemokine receptors (eg, interleukin [IL]-12, -15, -18) to aid in T-cell recruitment and DC navigation to lymphoid tissues (as reviewed by Steinman and Dhodapkar and Soling and Rainov ).
On localization to lymph organs rich in naive T cells, mature DCs present their processed antigens in a MHC-restricted manner. Through various interactions they are able to mobilize many different arms of the immune system, including CD8+ cytotoxic T cells (CTLs), CD4+ helper T cells, natural killer (NK) cells, and NK-like T cells. Each of these cell types plays an essential role in the antitumor response ( Fig. 2 ). T cells expressing CD8 coreceptors recognize and lyse tumor cells in an MHC-class I restricted fashion, and have received much of the credit as the primary effector cell in immunotherapy. CD4+ T cells have traditionally been known for their part in the expansion and maintenance of CD8+ CTLs, secretion of stimulatory cytokines, and the induction of lasting immunity. Their critical role in immunotherapy has become increasingly appreciated over the past few years, as studies have demonstrated that their absence may result in deficient DC maturation and CTL tolerance. Finally, NK and NK-like T cells have a unique niche in the leukocyte armament, being able to recognize and kill tumor cells that do not express surface markers, such as MHC-class I. Although the exact mechanism of recognition and elimination of tumor cells in the absence of MHC-restriction is yet to be elucidated, they serve as an important complement in the killing of tumor cells that may possess diminished surface marker presentation and avoid CTL detection.

Animal models
Preclinical animal models explored many of the methodologies and safety concerns regarding DC vaccines. In the late 1990s, Liau and colleagues were among the first to describe the effectiveness of DC vaccines in the antitumor immunity of gliomas using a rat model. Over the past decade, many groups have published similar studies with varying permutations in the choice of antigen, timing of vaccinations, and measures of therapeutic efficacy. The dissimilarities between study designs make it difficult to compare methodologies and their associated outcomes. Their value, however, lies within their ability to demonstrate the effectiveness of multiple different DC vaccine techniques in inducing antigen-specific cytotoxicity in vitro and in vivo. These studies have shown improved survival outcomes and the safety of the strategy.
Many of the initial animal studies were key in evaluating the effectiveness of DC vaccination techniques for tumors located in the “immunologically privileged” central nervous system (CNS). Antigen sources used included synthetic peptides ; acid-eluted tumor peptides ; tumor lysate ; DC-tumor fusion cells (FCs) ; and antigen-containing vectors, such as cDNA-RNA carrying viruses and tumor extract carrying liposomes. Many of these studies adopted strategies for antigen loading of DCs from previous experiments in peripheral neoplasms and initial treatment schedules were similarly based on regimens that showed promise in non-CNS immunotherapy. Nevertheless, the timing of DC administration variedly widely, with vaccinations being given before tumor inoculation, simultaneously with, or some time following tumor implantation. The number of vaccinations ranged from two to five times across the various studies.
Overall, most groups concluded that vaccination with antigen-pulsed DC was able to produce a significant antitumor immune response. This was evidenced by increased overall survival in rats and mice; greater degrees of T-cell infiltration (primarily CD8+) on histologic analysis of tumors; and more robust antitumor cytotoxicity assays when splenocytes were incubated with mouse glioma in vitro in immune responders. Although the studies conceded that pretumor vaccination resulted in greater survival in animal models, there are conflicting reports regarding the efficacy of DC vaccines when administered simultaneously or following tumor implantation. Groups reporting no improvement in survival with DC vaccination after an established tumor suggest that it may be caused by the immune system failing to generate an appropriate response quickly enough to counteract a rapidly growing tumor within a confined cranium. Studies in which T cell–mediated tumor killing was achieved showed, however, that the animals that did respond to DC vaccination obtained lasting antitumor memory, with significantly improved survival following tumor rechallenge compared with unvaccinated controls.
These animal studies played an important role in alleviating some of the concerns regarding DC immunotherapy for CNS tumors. Experimental allergic encephalomyelitis in particular was perhaps one of the most feared side effects, because previous studies have shown this lethal form of autoimmunity to occur following the injection of glioblastoma tissue into animals. Such signs of autoimmunity were not reported, however, in the more recent studies conducted to date. In addition to the information they provided regarding the applicability of different antigen sources and treatment schedules, the preclinical reports corroborated the idea that DC immunotherapy could be effectively used for intracranial neoplasms, and set the stage for further clinical studies.
Clinical trials
In 2000, Liau and colleagues published a case report on the first brain tumor patient to be treated with DC-based immunotherapy. A patient with histologically confirmed glioblastoma multiforme (GBM) received three biweekly intradermal injections of DCs pulsed with acid-eluted, allogeneic MHC-I matched GBM peptides. Although the authors were able to appreciate an immune response as evidenced by an increased infiltration of CD3+ T cells in postvaccination tumor, there was no objective clinical response from the treatment. The patient’s poor Karnofsky performance score in addition to the possible lack of antigen homology between the allogeneic GBM and the patient’s tumor may have contributed to the lack of clinical response or prolonged survival.
In a phase I dose-escalation clinical trial, 12 GBM patients were treated using DCs pulsed with autologous acid-eluted MHC tumor peptides in a dose-escalation study. Patients were separated into three cohorts, each receiving 1, 5, or 10 × 10 6 DCs per injection. Subjects tolerated the procedure well with no signs of autoimmunity. There were only minimal grade I toxicities related to the study vaccine, which were distributed similarly across all three dose groups. Although this study was not powered to measure efficacy, patients undergoing DC-based immunotherapy seemed to have an increased median time to progression (TTP) (15.5 months) and overall survival (23.4 months) compared with historical controls. Of note, tumor burden and disease progression at the time of vaccination was a critical determinant of systemic CTL activity, tumor infiltration by T cells, and overall survival. All patients who generated a systemic CTL response showed no MRI evidence of progressive disease at the time of vaccination. Conversely, no patient with actively progressive disease developed statistically significant cytotoxicity. Moreover, only patients possessing minimal tumor burden at the time of vaccination were found to have tumor-infiltrating lymphocytes (TILs) on postvaccination tissue examination. These findings suggest that active tumor progression or bulky residual burden can debilitate the initiation and propagation of an antitumor response. Interestingly, expression of the inhibitory cytokine transforming growth factor-β2 (TGF-β2) was found to be inversely proportional to the number of TILs found in tumor tissue following vaccination (IL-10 was not), implicating TGF-β2 as a possible mediator of immune evasion following vaccination. This study argues for the need for maximal resection or minimal residual disease to improve the efficacy of DC-mediated immunotherapy for glioma. Importantly, this clinical trial established the feasibility, safety, and immunologic potential of DC vaccines for brain tumor patients.
Yu and colleagues reported another phase I clinical trial using DC pulsed with autologous, acid-eluted peptides for glioma patients. In this study, nine patients with newly diagnosed malignant glioma received three biweekly subcutaneous injections of DCs loaded with acid-eluted tumor peptide. Systemic antitumor cytotoxicty was detected in four of the seven patients assessed; intratumoral CD8+ CTL and CD45RO+ memory T-cell infiltration was found in two of the four patients who underwent a second resection because of tumor progression. Patients receiving DC vaccination were found to have an increased median survival (455 days) compared with that of those in the control group (257 days).
Kikuchi and colleagues used DC-glioma FCs to vaccinate glioma patients in their phase I clinical trial. Eight patients with malignant gliomas received FCs intradermally every 3 weeks, with the total number of injections ranging from one to nine. An increased percentage of NK cells were found on FACS analysis in the peripheral blood of patients. In addition, an increase in IFN-γ release in peripheral blood mononuclear cells (PBMC) tumor coincubation with both autologous and allogeneic glioma was seen following DC vaccination. Two patients experienced a minor response and no serious side effects were observed. These findings suggested that nonspecific antitumor cytotoxicity may play a role in the DC-based immunotherapy of glioma.
Kobayashi and colleagues vaccinated five patients with autologous glioma RNA-pulsed DCs. They were able to demonstrate the presence of a strong CD8+ CTL response against autologous glioma accompanied by a weaker NK cell–mediated cytotoxicity in their patients. This finding was significant in three of the five patients treated. Notably, in the two patients with minimal immune responses, a constitutively increased expression of the inhibitory cytokine IL-10 and decreased expression of IFN-γ by CD8+ T cells was found in vitro.
Yamanaka and colleagues compared different routes of DC injection in their phase I-II clinical trial of 10 patients. DCs were pulsed with autologous tumor lysate and administered to patients intradermally (N = 5) or both intradermally and intratumorally by an Ommaya (N = 5) reservoir every 3 weeks for a total number of injections ranging from 1 to 10. Immunologically, they observed an increased percentage of NK cells and increased T cell–mediated antitumor activity. In addition, there was an increased intratumoral infiltration of CD4+ and CD8+ T cells in the two patients who underwent reoperation following vaccination. Radiographically, the two minor responses seen were in patients included in the combined intradermal-intratumoral administration group, suggesting that the additional intratumorally injected DCs may stimulate a more efficient antitumor immune response.
Wheeler and colleagues published a report examining the correlation between thymic function, as manifest through CD8+ recent thymic emigrant production, age, and patient outcome in 17 GBM patients undergoing DC immunotherapy. They found that thymic function, as reflected by its ability to produce CD8+ T cells, was directly proportional to good clinical outcomes in mice and human GBM patients and inversely proportional to age. Although patient age has long been a predictor of mortality and prognosis, their findings suggest that it is actually thymic function, which is inversely correlated with age, which may be the more telling factor. This nonspecific immune parameter may later serve as an important prognosticator in glioma immunotherapy.
Caruso and colleagues conducted a phase I study of nine pediatric brain tumor patients undergoing immunotherapy by autologous tumor RNA pulsed DCs. The cohort was comprised of a wide range of different tumor histologies ( Table 1 ). Although they detected a modest increase in antitumor antibodies in some patients, they did not appreciate any increase in T cell–mediated antitumor immunity. This may be explained by their findings that their patients had impaired immunocompetency before the start of the trial. Despite this, they reported clinical responses in three patients during the course of their study.
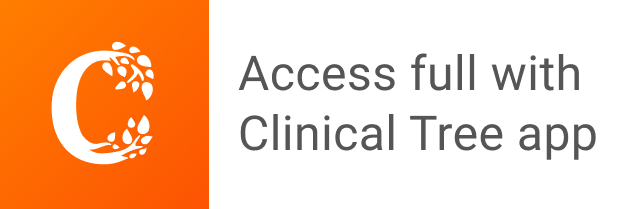