CLINICAL CASE | Spinal Cord Hemisection
A 21-year-old man suffered a gunshot injury. He was walking home from work with friends when he was hit by a stray bullet. He was unconscious when the ambulance arrived. When he regained consciousness in the emergency room, he reported that he was unable to move his right foot and that his right leg felt numb.
Neurological and radiological examination revealed that the bullet entered at about the mid-thoracic level. There was complete loss of right lower limb motor function and loss of tactile sensation (as well as other mechanosensations, including position sense and vibration sense), also on the right, at T10 and below. Pain sensation was examined with pin prick, and testing revealed an absence of pain on the left leg only, extending rostrally to T11. Figure 10–1A shows the distribution of sensory loss.
Answer the following questions based on your reading of this chapter, as well as review of Chapters 4 and 5 on mechanosensations and pain.
1. Why are pain and tactile sensations impaired on opposite sides of the body?
2. Why does the patient have preserved pain but not touch sensation over the T11 dermatome?
3. Explain why the leg on the side with the tactile sensation impairment is paralyzed, but not paralyzed on the side without pain?
Key neurological signs and corresponding damaged structures Alternate distributions for pain and tactile sensory lossThe lesion damages two distinct populations of ascending somatic sensory fibers. Damaged ascending pain fibers ascend to the lesion site after crossing the midline caudal to the lesion. Damaged ascending tactile fibers ascend to the lesion site without decussating; they cross the midline rostral to the lesion, in the medulla. By following the pain and tactile circuits back to their origins in the periphery, the different sides of sensory loss are revealed.
Preserved pain sense one to two segments caudal to tactile lossPain is perceived down to a more caudal level than tactile stimuli. Pain fibers cross the midline over multiple spinal levels (ie, they cross as they ascend). The first pain fibers to become damaged have entered the spinal cord one to two segments below the lesion (Figure 10–1A; see also Figure 5–1). Pain fibers entering at the level of the injury will bypass the lesion. It should be noted that the spinal injury can damage entering primary afferents themselves, and the nearby dorsal horn, and would be expected to produce a small amount of sensory loss just at the dermatomal level on the side of the lesion. However, because of dermatomal overlap (see Figure 4–5), this will be minimized and may even go unnoticed.
Ipsilateral motor and tactile lossThe corticospinal tract decussates in the medulla (see Figure 10–4A). For this reason, the lesion interrupts the descending pathway controlling muscles on the same side (Figure 10–1A).
FIGURE 10–1
Spinal hemisection produces paralysis and a loss of mechanosensations caudal to, and on the same side as, the lesion. This injury produces a loss of pain, temperature, and itch caudal to, and on the opposite side as, the lesion A. Circuit changes associated with spinal cord hemisection. B. Pattern of somatic sensory loss in the patient. Pain is lost on his left side (orange); tactile sensations are lost on the right side (green). Three key pathways are affected. (1) The mechanoreceptive pathway, the dorsal columns, is interrupted ipsilateral to its origin. (2) The pain pathway, the anterolateral system, is interrupted contralateral to its origin. (3) The motor pathway, the corticospinal tract, is interrupted ipsilateral to its target motor neurons and muscle.

The motor systems of the brain and spinal cord work together to control body movements. These systems must fulfill diverse tasks because the functions of the muscles of the body differ markedly. Consider, for instance, the fine control required of hand muscles when grasping a china cup, in contrast to the gross strength required of back and leg muscles in lifting a box full of books. The muscles that move the eyes have an entirely different set of tasks, such as positioning the eyes to capture information from the visual world. The principal function of facial muscles is not movement but rather creating facial expressions as well as assisting in speech articulation. These jobs are so varied that it is not surprising that the motor systems have many specific components devoted to the control of different motor functions.
The motor systems are clinically very important because damage, depending on the location and severity, produces wide-ranging impairments in strength, volition, and coordination. Profound weakness or paralysis after a stroke or a traumatic spinal cord injury limits an injured person’s capacity for independence. Disordered coordination can make control of simple daily activities, such as tying a knot or eating, impossible, requiring assistance by a care giver. Defective eye movement control can impair cognitive functions, such as a reduced capacity to read text or discern the locations of objects.
The first three motor system chapters examine the components that connect higher control centers with motor neurons; these motor systems are essential for contracting muscle. This chapter focuses on the neuroanatomy of descending spinal cord pathways and spinal cord circuits for limb control and posture. Next, the pathways that control facial and other head muscles are discussed in Chapter 11. Because eye movement and balance share many neural circuits, and a strong interrelationship with the vestibular system, these topics are covered jointly in Chapter 12. The last two motor system chapters focus on important behavioral integrative centers: the cerebellum (Chapter 13) and basal ganglia (Chapter 14).
Four separate components of the central nervous system together control skeletal muscles of the limbs and trunk muscles for posture (Figure 10–2): (1) descending motor pathways, together with their associated origins in the cerebral cortex and brain stem, (2) spinal motor circuits, including motor neurons and interneurons, (3) basal ganglia (yellow), and (4) the cerebellum.
FIGURE 10–2
General organization of the motor systems. Center. General organization of the motor systems. There are four major components to the motor systems: descending cortical and brain stem pathways, motor neurons and interneurons of the spinal cord, basal ganglia, and cerebellum. Both the basal ganglia and cerebellum influence movements through connections to the cortical and brain stem motor pathways. Upper inset. Key cortical regions for controlling movement. The limbic and prefrontal association areas are involved in the initial decision to move, in relation to motivational and emotional factors. In reaching to grasp an object, the visual areas process information about the location and shape of the object. This information is transmitted, via the “where” or “action” path first to the posterior parietal lobe, and then to the premotor areas, which are important in movement planning. From there, information is transmitted to the primary motor cortex, from which descending control signals are sent to the motor neurons.

The regions of the cerebral cortex and brain stem that contribute to the descending motor pathways are organized much like the ascending sensory pathways but in reverse: from the cerebral cortex or brain stem to the muscles of our limbs and trunk. The brain stem motor pathways engage in relatively automatic control, such as rapid postural adjustments and in-flight correction of misdirected movements. By contrast, the cortical motor pathways participate in more refined, flexible, and adaptive control, such as reaching to objects, grasping, and tool use.
Motor neurons and interneurons comprise the spinal motor circuits, the second component of the limb and posture control systems. The motor pathways synapse directly on motor neurons as well as on interneurons that in turn synapse on motor neurons (Figure 10–2). For muscles of the limbs and trunk, motor neurons and most interneurons are found in the ventral horn and intermediate zone of the spinal cord (Figure 10–3). The intermediate zone corresponds primarily to the spinal gray matter lateral to the central canal. It is sometimes included within the ventral horn. For muscles of the head, including facial muscles, the motor neurons and interneurons are located in the cranial nerve motor nuclei and the reticular formation (see Chapter 11). The spinal motor circuits are not only the target of the descending motor pathways but also can function relatively independently through their reflexive and intrinsic motor actions. The simplest reflex is the monosynaptic stretch reflex (see Figure 2–6). More complex, polysynaptic, reflexes produce automatic limb withdrawal from a noxious stimulus as well as postural reflexes. Stepping patterns are organized by spinal circuits within the lumbar and sacral segments. When motor pathways synapse directly on motor neurons, they are able to activate individual muscles and even groups of muscle fibers within a muscle. This is because a single motor neuron connects to a limited set of fibers within one muscle, termed the motor unit. By connecting to interneurons in spinal circuits, a motor pathway is able to regulate motor reflex actions. For example, if you grasp an unexpectedly hot teacup that is a family heirloom, you may not want to reflexively release your grip, for risk of breaking the cup. Activating muscles through interneurons, rather than more directly through motor neurons, may also enable a motor pathway to select a group of muscles that have a complex behavioral action, such as stepping.
FIGURE 10–3
Somatotopic organization of the ventral horn and motor pathways. Schematic diagram of the spinal cord, showing the somatotopic organization of the ventral horn and indicating the general locations of motor neurons innervating limb and axial muscles and flexor and extensor muscles. A partial homunculus is superimposed on the ventral horns. (Adapted from Crosby EC, Humphrey T, Lauer EW. Correlative Anatomy of the Nervous System. New York, NY: Macmillan; 1962.)

The third and fourth components of the motor systems, the cerebellum and the basal ganglia (Figure 10–2; see Chapters 13 and 14), do not contain neurons that project directly to spinal motor circuits. Nevertheless, these structures have a powerful regulatory influence over motor behavior. They act indirectly in controlling motor behavior through their effects on the descending brain stem pathways and, via the thalamus, cortical pathways (Figure 10–2). The specific contributions of the cerebellum and basal ganglia to movement control are surprisingly elusive despite centuries of study. The cerebellum is part of a set of neural circuits for ensuring that movements are precise and accurate. However, this description only captures a small part of cerebellar function; the cerebellum has additional nonmotor functions. The specific contributions of the basal ganglia to normal motor action are largely unknown. However, we are well aware of how movements become disordered when the basal ganglia are damaged. For example, in patients with Parkinson disease, a neurodegenerative disease that primarily affects the basal ganglia, movements are slow or fail to be initiated and patients have significant tremor. Like the cerebellum, the basal ganglia have many nonmotor functions.
Myriad areas of the cerebral cortex provide the motor systems with information essential for making accurate movements. For example, during visually guided movements—such as reaching to grasp a cup—the process of translating thoughts and sensations into action begins with the initial decision to move. This process is dependent on the cortical limbic and prefrontal association areas (Figure 10–2; brain inset upper left), which are involved in emotions, motivation, and cognition. The magnocellular visual system processes visual information about the locations of objects for guiding movements. It provides the major visual input to the “where” or “action” pathway (Figure 10–2, inset; see Figures 7–15 and 7–16). The magnocellular visual system projects to the posterior parietal cortex, an area important for identifying the location of salient objects in the environment and for directing our attention to these objects (Figure 10–2). Visual information next is distributed to certain premotor areas of the frontal lobe, where the plan of action to reach for the cup is formed, a plan that specifies the path the hand takes to reach the object and prepares the hand for grasping or manipulation once contact occurs. The next step in translating into action the decision to reach is directing muscles to contract. This step primarily involves the corticospinal tract, which originates from both the premotor areas and the primary motor cortex. The corticospinal tract transmits control signals to motor neurons and to interneurons (Figure 10–2). The cortical motor pathways also recruit brain stem pathways for coordinating voluntary movements with postural adjustments (Figure 10–2), such as maintaining balance when lifting a heavy object.
Descending pathways serve a diversity of functions. We recognize their principal function in controlling movements because when they become damaged, people become weak or paralyzed. However, in addition to movement control, the descending pathways regulate somatic sensory processing and the autonomic nervous system. Whereas the motor control functions are mediated by synaptic connections with motor neurons and interneurons, the somatic sensory functions are associated with connections on dorsal horn neurons and somatic sensory relay nuclei in the brain stem. We have already learned about one somatic sensory regulatory function: the pain suppression function of the raphespinal pathway (see Chapter 5). The descending pathways synapse on preganglionic autonomic neurons in the brain stem and spinal cord to regulate autonomic nervous system functions. The autonomic control pathways are examined in Chapter 15 with the autonomic nervous system. Finally, the descending pathways also can influence plasticity of spinal circuits during motor learning and have important trophic functions during development. The pathways from the cerebral cortex serve all of these functions. It is not known if the same applies to all of the brain stem pathways. Here we will focus on the motor control functions of the descending pathways.
Seven major descending motor control pathways terminate in motor centers of the brain stem and spinal cord (Table 10–1). Three of these pathways originate in layer V of the cerebral cortex, primarily in the frontal lobe: (1) the lateral corticospinal tract, (2) the ventral (or anterior) corticospinal tract, and (3) the corticobulbar tract. The corticobulbar tract terminates primarily in cranial motor nuclei in the pons and medulla and is the cranial equivalent of the corticospinal tracts; it will be considered in Chapter 11. Collectively, these cortical pathways participate in controlling the most adaptive and flexible movements, such as finger control during tool use, regulating posture during limb movements, and during speech. The remaining four pathways originate from brain stem nuclei: (4) the rubrospinal tract, involved in automatic limb control; (5) the reticulospinal tracts, which participate in automatic control of proximal muscles and locomotion; (6) the tectospinal tract, involved in coordinating head movements with eye movements; and (7) the vestibulospinal tracts, critical for maintaining balance. There are also neurotransmitter-specific descending pathways (see Chapter 2) that originate from the serotonergic raphe nuclei, noradrenergic locus ceruleus, and dopaminergic neurons of the midbrain and diencephalon, all of which have extensive spinal cord terminations. In addition to pain regulation, the actions of these descending pathways can rapidly regulate both the strength of muscle contraction and reflexes.
Tract | Site of origin | Decussation | Spinal cord column | Site of termination | Spinal/brain stem level of termination | Function |
---|---|---|---|---|---|---|
Cerebral Cortex Corticospinal | ||||||
Lateral | Areas 6, 4, 1, 2, 3, 5, 7, 23 | Crossed—pyramidal Decussation | Lateral | Dorsal horn, intermediate zone, ventral horn | All levels | Sensory control, voluntary movement (limb muscles) |
Ventral | Areas 6, 4 | Uncrossed1 | Ventral | Intermediate zone, ventral horn | Upper cervical | Voluntary movement (axial muscles) |
Corticobulbar | Areas 6, 4, 1, 2, 3, 5, 7, 23 | Crossed and uncrossed2 | Brain stem only | Cranial nerve sensory and motor nuclei, reticular formation | Midbrain, pons, medulla | Sensory control, voluntary movement (cranial muscles) |
Brain Stem | ||||||
Rubrospinal | Red nucleus (magnocellular) | Ventral tegmentum | Lateral | Lateral intermediate zone, ventral horn | All levels | Voluntary movement, limb muscles |
Vestibulospinal | ||||||
Lateral | Lateral vestibular nucleus | Ipsilateral1 | Ventral | Medial intermediate zone, ventral horn | All levels | Balance |
Medial | Medial vestibular nucleus | Bilateral | Ventral | Medial intermediate zone, ventral horn | Upper cervical | Head position/neck muscles |
Reticulospinal Pontine | All levels | |||||
Pontine reticular formation | Ipsilateral1 | Ventral | Medial intermediate horn, ventral horn | All levels | Automatic movement, axial, and limb muscles | |
Medullary | Medullary reticular formation | Ipsilateral1 | Ventrolateral | Medial intermediate zone, ventral horn | All levels | Automatic movement, axial, and limb muscles |
Tectospinal | Deep superior colliculus | Dorsal tegmentum | Ventral | Medial intermediate zone, ventral horn | Upper cervical | Coordinates neck with eye movements |
Indirect Cortical Pathways | ||||||
Coritcorubrospinal | Frontal lobe → red nucleus | |||||
Corticoreticular | Frontal lobe → reticular formation | |||||
Corticovestibular | Parietal lobe → vestibular nuclei |
How are the actions of the myriad descending motor pathways coordinated during movement? What is the logic of their organization? By taking a combined clinical, anatomical, and physiological perspective, three principles governing the organization of the motor pathways emerge.
The functional organization of the descending pathways parallels the somatotopic organization of the motor nuclei in the ventral horn
The motor neurons innervating limb muscles, and the interneurons from which they receive input, are located in the lateral ventral horn and intermediate zone. In contrast, motor neurons innervating axial and girdle muscles (ie, neck and shoulder muscles), and their associated interneurons, are located in the medial ventral horn and intermediate zone. The mediolateral somatotopic organization of the intermediate zone and ventral horn is easy to remember because it mimics the form of the body (Figure 10–3). This mediolateral somatotopic organization also applies to the location of the descending motor pathways in the spinal cord white matter (discussed in detail below). The pathways that descend in the lateral portion of the spinal cord white matter control limb muscles. In contrast, the pathways that descend in the medial portion of the white matter control axial and girdle muscles.
Movements are controlled by direct cortical projections to the spinal cord and indirect cortical projections via brain stem nuclei
The lateral and ventral corticospinal tracts can control spinal circuits through their direct spinal projections. In addition, the cortical motor regions project to brain stem nuclei that give rise to motor pathways: the red nucleus, superior colliculus, reticular formation, and vestibular nuclei. This is shown in Figure 10–2 (center) as an arrow connecting the cortical pathway with the brain stem. Thus, the cerebral cortex can also influence movements through indirect brain stem connections. For example, the two components of the corticoreticulo-spinal pathway are the cortical projection to particular nuclei of the reticular formation and then the reticulospinal tract’s projection to the spinal cord. Considering all combinations of pathways, the projection neurons of the cerebral cortex constitute the highest level in the hierarchy, the brain stem projection neurons comprise the next lower level, and spinal interneurons and the motor neurons are the two lowest levels. Study of patients with motor pathway damage suggests that direct spinal connections mediate more precise joint control, such as our ability to move one finger independent of the others (termed fractionated control), whereas the indirect connections serve coarser control functions, such as moving all fingers together during a powerful grasp. Can brain stem motor pathways work independent of the cortical pathways? The answer is probably yes, based on laboratory animal studies. Both the cerebellum and basal ganglia have direct connections with brain stem motor pathways (Figure 10–2), which could influence brain stem motor pathway function without cortex involvement. A challenge for promoting recovery of motor function after cortical damage, such as a stroke, is to foster a more effective independent brain stem control.
Typically the axon of a descending projection neuron, in addition to making monosynaptic connections with motoneurons, makes monosynaptic connections with spinal cord interneurons (Figure 10–2, bottom). Depending on the particular interneuron type (see Figure 10–16A), the interneurons have different functions. Some interneurons receive input from somatic sensory receptors for the reflex control of movement. For example, particular interneurons receive input from nociceptors and mediate limb withdrawal reflexes in response to painful stimuli, such as when you jerk your hand away from a hot stove. Other interneurons coordinate the left-right limb motor actions during walking, while others still are important for upper limb-lower limb coordination.
The lateral corticospinal tract and the rubrospinal tract (Table 10–1) are the two lateral motor pathways. The neurons that give rise to these pathways have a somatotopic organization. Moreover, the lateral corticospinal and rubrospinal tracts control muscles on the contralateral side of the body. The lateral corticospinal tract is the principal motor control pathway in humans. A lesion of this pathway anywhere along its path to the motor neuron, such as after a stroke in the subcortical white matter, produces devastating and persistent limb use impairments. Box 10–1 discusses the major changes in motor and reflex function after damage to the corticospinal system. There is a loss of voluntary control and weakness, termed paresis, that can make standing impossible without assistance. There is also a loss of the ability to move one finger independent of the others, which is termed fractionation. Manual dexterity depends on fractionation, without which hand movements are clumsy and imprecise. Loss of corticospinal tract control after stroke is the most common cause of paralysis.
Box 10–1 Lesions of the Descending Cortical Pathway in the Brain and Spinal Cord Produce Flaccid Paralysis Followed by Changes in Spinal Reflex Function
Lesions involving the posterior limb of the internal capsule, ventral brain stem, and spinal cord isolate motor neurons from voluntary motor control signals transmitted by the descending motor pathways. This produces a common set of motor signs. Initially these signs include flaccid paralysis and reduced muscle reflexes (eg, knee-jerk reflex). Clinical examination also reveals decreased muscle tone, signaled by the marked reduction in resistance felt by the examiner to passive movement of the limb. These are the classic signs of weakness and, if sufficiently severe, paralysis. These signs are attributable largely to interruption of the corticospinal fibers even though the corticoreticular and corticopontine fibers are damaged. The laterality of the signs depends on whether the lesion occurs in the brain or the spinal cord. Spinal cord injury is particularly devastating because all muscles of the body caudal to the level of injury can become affected.
With time after the lesion, a similar examination often reveals increased muscle tone and exaggerated muscle stretch (or myotatic) reflexes, termed hyperreflexia. The increased muscle tone is due to increased reflex activity when the examiner passively stretches the limb. It is not known what causes hyperreflexia; the cause may not be the same after all types of injury. It has been suggested that damage of the indirect corticoreticular pathway may lead to a loss of inhibitory signals to reflex centers in the spinal cord. This could lead to an increase in reflexes, through disinhibition. Long-term synaptic plasticity of spinal reflex circuits may also play an important role in the delayed time course of this effect. After spinal cord injury, or damage to descending pathways, motor neurons change their intrinsic properties and become more excitable.
In addition to producing hyperreflexia, lesions of the corticospinal tract result in the emergence of abnormal reflexes, the most notable of which is Babinski’s sign. This sign involves extension (also termed dorsiflexion) of the big toe in response to scratching the lateral margin and then the ball of the foot (but not the toes). Babinski’s sign is thought to be a withdrawal reflex. Normally such withdrawal of the big toe is produced by scratching the toe’s ventral surface. After damage to the descending cortical fibers, the reflex can be evoked from a much larger area than normal. Interestingly, the Babinski’s sign is normally present in young children, before maturation of the corticospinal tract. Hoffmann’s sign, which is thumb adduction in response to flexion of the distal phalanx of the third digit, is an example of an abnormal upper limb reflex caused by damage to the descending cortical fibers. Vascular lesions are a common cause of damage to the descending motor pathways, thereby affecting both direct and indirect corticospinal projections (see Clinical Case, Chapter 11).
The major site of origin of the lateral corticospinal tract is the primary motor cortex (Figure 10–4A, inset), although axons also originate from the premotor cortical regions (supplementary motor area, cingulate motor area, and premotor cortex) and the somatic sensory cortical areas. Descending axons in the tract that originate in the primary motor cortex course within the cerebral hemisphere in the posterior limb of the internal capsule and, in the midbrain, in the basis pedunculi (Figure 10–4A). Next on its descending course, the tract disappears beneath the ventral surface of the pons only to reappear on the ventral surface of the medulla as the pyramid. At the junction of the spinal cord and medulla, most of the axons decussate (pyramidal decussation) and descend in the dorsolateral portion of the lateral column of the spinal cord white matter; hence the name lateral corticospinal tract (see Figure 10–6). This pathway terminates primarily in the lateral portions of the intermediate zone and ventral horn of the cervical and lumbosacral cord, the locations of neurons that control the arm and leg. There is a contingent of axons that terminate on the ipsilateral side. Some of these axons descend ipsilaterally; many descend contralaterally and re-cross the midline within the gray matter in lamina 10. The function of these ipsilateral axons is not well understood. After unilateral damage to the lateral corticospinal tract, the ipsilaterally terminating axons may contribute to recovery of some motor functions.
FIGURE 10–4
Laterally descending pathways. A. Lateral corticospinal tract. The inset shows the locations of the primary motor cortex and three premotor areas: the supplementary motor area, the cingulate motor areas, and the premotor cortex. The lateral corticospinal tract also originates from neurons located in area 6 and the parietal lobe. Note the branch into the red nucleus; this is the corticorubro component of the indirect corticorubrospinal path. B. Rubrospinal tract.
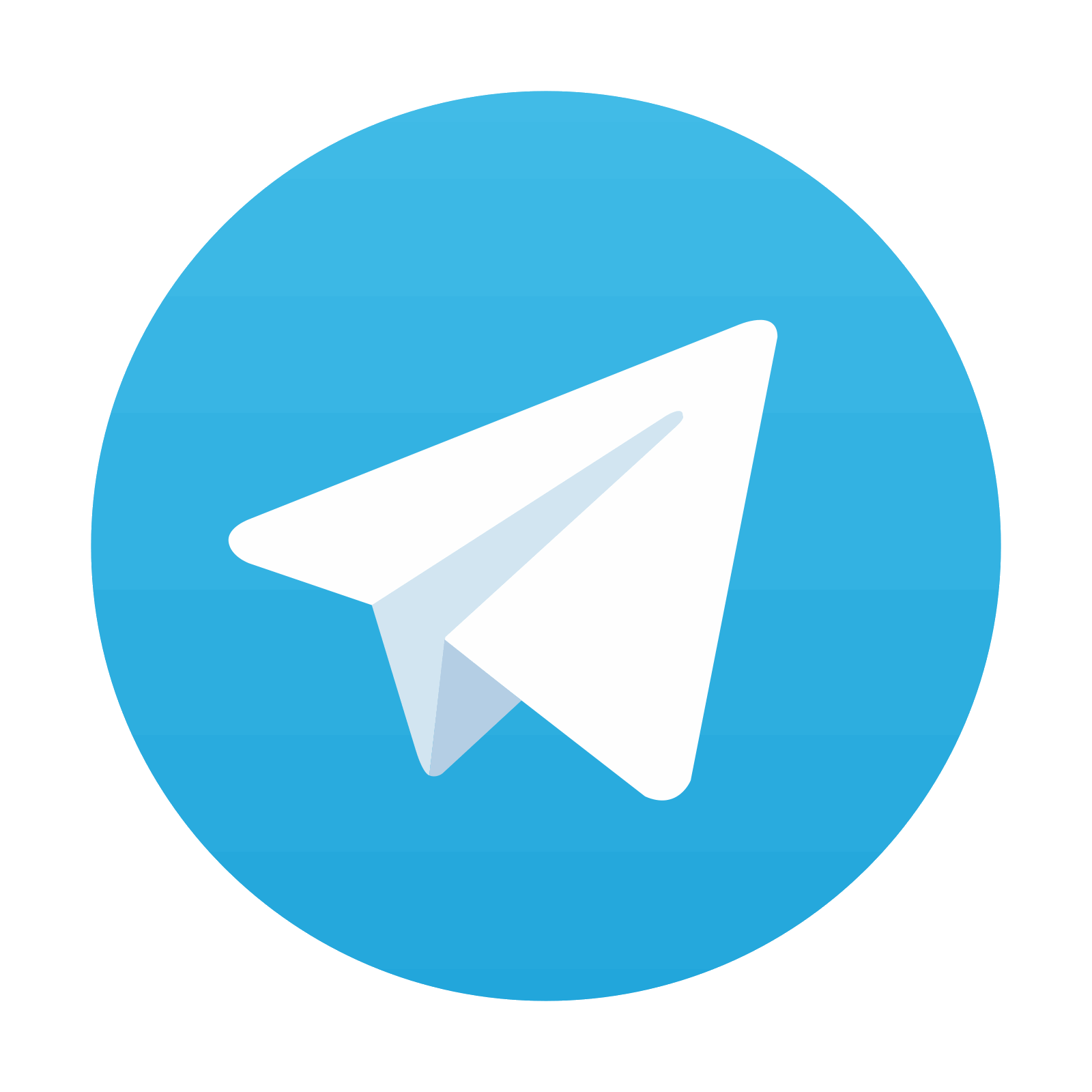
Stay updated, free articles. Join our Telegram channel
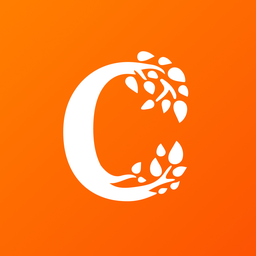
Full access? Get Clinical Tree
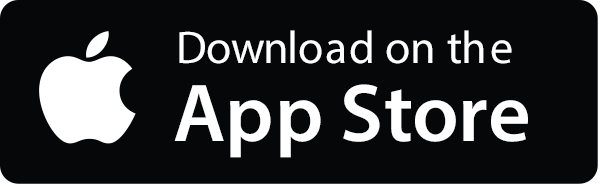
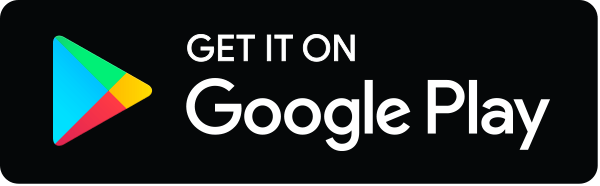
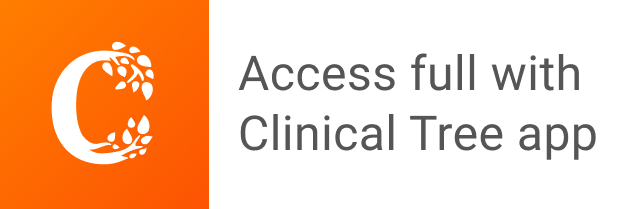