(1)
Cyclotron Research Centre, University of Liège, Allée du 6 Août, 8, 4000 Liège, Belgium
Keywords
Neuroimaging techniquesStructural neuroimagingPositron emission tomographyFunctional magnetic resonance imagingSleepSleep disordersIntroduction
During the last two decades, neuroimaging techniques have been applied to sleep studies and contributed to a better understanding of sleeping process and its disorders. These techniques can provide information about both brain structure and function. This chapter reviews both aspects in the field of sleep research. At first, we will give an overview of the different imaging techniques and findings they brought in normal human sleep. A short paragraph will then be dedicated to imaging studies of sleep and memory. Finally, we will finish with a section on neuroimaging in sleep disorders. Further information can be found in previous reviews on the same topic.
1.
Maquet P. Functional neuroimaging of normal human sleep by positron emission tomography. J Sleep Res. 2000 Sept;9(3):207–31.
2.
Desseilles M, Dang-Vu T, Schabus M, Sterpenich V, Maquet P, Schwartz S. Neuroimaging insights into the pathophysiology of sleep disorders. Sleep. 2008 Jun;31(6):777–94.
3.
Dang-Vu TT, Schabus M, Desseilles M, Sterpenich V, Bonjean M, Maquet P. Functional neuroimaging insights into the physiology of human sleep. Sleep. 2010 Dec;33(12):1589–603.
4.
Jedidi Z, Rikir E, Muto V, Mascetti L, Kussé C, Foret A, Shaffii-Le Bourdiec A, Vandewalle G, Maquet P. Functional neuroimaging of the reciprocal influences between sleep and wakefulness. Pflugers Arch. 2012 Jan;463(1).
Neuroanatomical Assessments
Structural brain imaging is clinically relevant for two reasons. First, it is widely available and second, it requires a limited collaboration from the patient. The objective is usually to characterize regional-specific modifications in brain structure between healthy participants and patients suffering from certain sleep disorders. These cerebral changes are supposed to be relatively persistent and thus can be observed independently from the patient’s state of vigilance during the examination. It does not necessarily require the patient to be sleeping (or performing a task).
Within this type of neuroanatomical ways of assessment, the two basic techniques are Voxel-based morphometry (VBM) and magnetic resonance spectroscopy (MRS).
VBM is becoming the standard way of analyzing structural brain data. Based on high resolution scans, VBM allows between-group, statistical comparisons of tissue composition (gray and white matter) across all brain regions. Usually built on a general linear model, it tests for voxel-wise differences in signal between patients and controls or for linear regression between structural brain aspects and specific explanatory variables (age, duration of disease…).
MRS allows obtaining biochemical information about regional brain tissue composition by measuring absolute and relative rate of different compounds such as choline (Cho), creatinine (Cr), N-acetyl aspartate (NAA) .
As VBM and MRS exclusively concern pathological sleep research we will reserve illustrations of their applications for the last section dedicated to neuroimaging in sleep disorders.
Sleep Functional Neuroimaging
In contrast to structural imaging, functional imaging offers a dynamical approach to probe behavioral states, such as sleep and wakefulness . As it confers a better and faster temporal resolution, it tracks down fluctuations in the global and/or regional brain activity. Sleep functional neuroimaging by definition implies the assessment of a concomitant state of vigilance, and consequently requires simultaneous EEG recordings.
Initially, sleep functional imaging studies used positron emission tomography (PET) and single photon emission computed tomography (SPECT). However, during the last decade, functional magnetic resonance imaging (fMRI) has emerged as the “gold” technique to probe regional brain activity during sleep, despite the difficulty inherent to simultaneous EEG and fMRI acquisitions [1].
Global Metabolism Level During Sleep: First Studies Using 18-FDG PET
Glucose metabolism , determined by [18F] fluorodeoxyglucosed (18-FDG), was the 1980s most popular marker of brain activity measured by PET. Buchsbaum et al. and Maquet et al. were the first to apply this method in sleep research by two pioneering studies, carried out in 1989 and 1990 respectively. They showed that global glucose metabolism was lower during slow wave sleep (SWS), while it was sustained during REM sleep as compared to wakefulness [2, 3].
18-FDG PET studies rapidly found their limits in sleep research. The poor spatial resolution of this method (~5 mm) and the absence of voxel-wise analysis techniques limited an in-depth characterization of regional brain function. However, an activation of left temporal and occipital areas during REM sleep and a bilateral thalamic deactivation during slow wave sleep (SWS) were already reported [3, 4].
Another 18-FDG drawback is its very limited time resolution. Its long time acquisition (45 min) restricts studies to long lasting effects such as wakefulness, REM and NREM sleep episodes, although the long half-life of 18-FDG (108 min) limits the repetition of measurements in the same subject in a single session [5]. The latter advent of 15-oxygen labeled water (H2 15O) in PET studies came as a major progress.
First Assessments of Regional Cerebral Activity: PET with Infusions of H2 15O
After using 18-FDG PET, sleep researchers, including Hofle (1997), Andersson (1998), and Maquet and Phillips (1998), started to investigate regional cerebral activity by means of PET with infusions of 15-oxygen labeled water (H2 15O). In contrast to 18-FDG PET, H2 15O studies do not measure glucose metabolism , but rather the regional cerebral blood flow (rCBF). The reduced time acquisition (1.5 min) and labeled compounds/shorter half-life (123 s) significantly improved time resolution [5].
During NREM sleep (NREMS), H2 15O PET studies showed a global but also regional reductions of brain activity in cortical (prefrontal, anterior cingulate, precuneus, associative parietal, and mesial aspect of temporal lobe) and subcortical (brainstem, thalamus, basal ganglia, hypothalamus, basal forebrain) regions [6, 7]. These areas include neuronal populations involved in arousal and awakening, which are among the most activated regions during wakefulness.
Using H2 15O PET, REM sleep has been associated with the activation of pontine tegmentum, basal forebrain, thalamus, limbic areas (amygdala, hippocampus, anterior cingulate cortex), and temporo-occipital cortices whereas associative prefrontal and parietal areas were deactivated. This pattern can readily be associated with dream features, which mostly occur during REM sleep. Visual and auditory dream perception can be, respectively, correlated to occipital and temporal activations, while affect and emotional intensification can be related to limbic and paralimbic system activation. Conversely, the quiescence of prefrontal areas may account for temporal distortions, weakening of self-reflecting control, or amnesia on awakening [8–11].
Despite the advantages brought on by H2 15O, PET temporal resolution could not directly capture changes in brain activity during transient events, such as a spindle or a slow wave. However, attempts were made to correlate rCBF variations with EEG spectral activity in sigma (spindles) and delta (slow wave activity) ranges. Accordingly, sigma power (12–16 Hz) was negatively correlated to rCBF in the thalamus bilaterally [12] indicating its central role in spindle generation. In a similar vein, delta power (0.5–4 Hz) was negatively correlated with rCBF in several brain areas such as thalamus, cerebellum, anterior and posterior cingulate gyrus, precuneus, orbitofrontal cortex, ventro medial prefrontal cortex (vMPFC), basal forebrain, striatum (putamen), and insula [13]. This mapping shows striking similarities with the distribution of deactivated brain areas during NREM sleep, as compared to wakefulness, suggesting a similar neural network in the regulation of NREM sleep and slow waves. The strongest association with delta power was found in vMPFC correlating with the prefrontal predominance of slow wave activity observed in EEG recordings.
Functional Magnetic Resonance Imaging
Functional MRI describes neural activity by assessing the blood oxygen level-dependent (BOLD) signal, a non-linear mix of changes in local brain vascular volume, blood flow, and level of deoxy-hemoglobin. Its success benefits from better spatial and temporal resolution, relative to emission tomography . The improvement of the latter compared to PET has enabled the direct observation of changes in brain activity for short-lasting events, such as a spindle or a slow wave. In contrast to PET, fMRI is X-ray free and completely non-invasive since it requires neither catheter nor radioactive compound injection. However, the high noise level and exiguity of the device make the environment rather unfavorable to sleep. The EEG recording is also made difficult by the magnetic environment, resulting mainly in gradient scan and pulse-related artifacts which have required the development of MRI compatible EEG caps and artifacts rejecting processes [14]. For comparison between PET and fMRI refer to Table 64.1.
Table 64.1
As indicated in Table 64.1, each technique has its own advantages and drawbacks in terms of spatial and temporal resolutions but feasibility, accessibility, safety, and cost
PET | fMRI | |
---|---|---|
What it shows | Distribution of compounds labeled with positron-emitting isotopes | Variations in brain perfusion related to neural activity by assessing the blood oxygen level-dependent signal (BOLD) |
Time resolution | Depends on labeled compounds with which vary the biological half-life (HF) and the required exam time acquisition (TA) | ~ 10 s |
FDG | ||
TA: 45 min: description restricted to long lasting changes | ||
long half-life (108 min): restrict the repetition of measurements of a same subject in a single session | ||
H2 15O | ||
shorter half-life (123 s) | ||
time acquisition (1–2 min) | ||
Spatial resolution | ~ 5 mm | ~ 2–3 mm |
Comfort | Require catheterism | Narrow space and noise hamper |
Safety | Infectious risk due to catheterism,; radioactive agent injection, X-ray exposition | Totally non invasive, no injection of a radioactive agent, no irradiation; respect of ferromagnetic contraindications and precautions |
Cost | Important infrastructure required (cyclotron and chemists for radioactive compound production, …) | |
EEG combining | No compatibility or artifacts problems | Requires an MRI compatible EEG cap and amplifier Post processing necessary to remove scan gradient and cardio ballistic artifacts |
Spatial patterns of regional brain activity described in fMRI during NREMS were globally consistent with those reported by PET sleep studies. However, fMRI allowed to address NREMS phasic activity and was thus able to report transient brain activations while PET studies consistently reported decreased brain activity [15].
NREM phasic activities, as assessed by fMRI studies, are associated with increased (but not decreased) brain responses. For instance, spindles are positively correlated with increased activity in lateral and posterior aspects of the thalamus, paralimbic (anterior cingulate cortex, insula), and neocortex (superior temporal gyrus). This confirms the thalamic involvement in spindles generation and suggests the participation of specific cortical areas in their modulation [16]. Likewise, slow waves are associated with significantly increased activity in inferior and medial frontal cortices, parahippocampal gyrus, precuneus, posterior cingulate cortex, ponto-mesencephalic tegmentum, and cerebellum. These results contrast with the classical view of brainstem nuclei promoting vigilance and wakefulness, because it suggests that several pontine structures including the locus coeruleus might be active during NREM sleep concomitant with SWA [15]. Cortical responses during slow wave occur in brain areas which are now known as major hubs in cortical structural connectivity and are also the most active during wakefulness [17]. These results have now been replicated [18] and are supported by source reconstruction of slow waves [19]. Altogether, these data underline that simple reduction of NREMS to a state of global and regional brain activity decrease is no longer defensible. See Fig. 64.1 for fMRI neural correlates of NREM sleep oscillations.
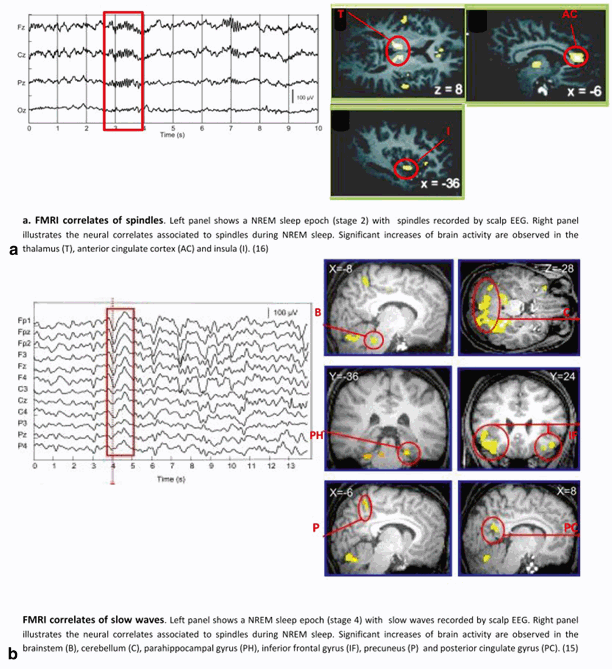
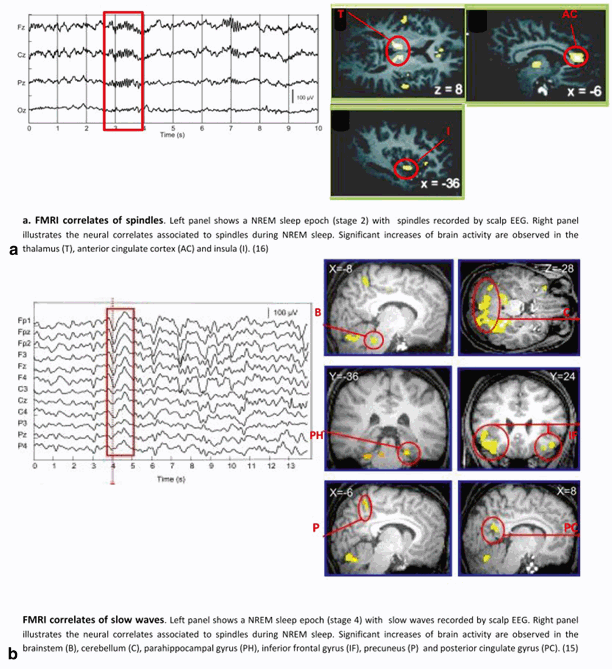
Fig. 64.1
Neural correlates of NREM sleep oscillations as assessed by EEG/fMRI
To date, REM sleep has been much less investigated by fMRI studies. Positive correlation between BOLD signal and density of REM was reported in thalamus, pons, and primary visual cortex, which is the main recording site of ponto-geniculo-occipital (PGO) activity. Activations described in the anterior cingulate cortex, parahippocampal gyrus, and amygdala make these regions potentially involved in REM sleep modulation [20, 21].
Sleep and Memory
Sleep is considered to have life-sustaining functions. In particular, it is now suggested that sleep intimately results from the energy metabolic demands implied by synaptic transmission induced by wakefulness [22]. It has also been associated with consolidation of recent memories. Recent reviews are available on these topics [23–25]. This very brief section aims to illustrate the interest of functional neuroimaging in better understanding these processes.
PET and fMRI have shown that waking experience influences regional brain activity during subsequent NREM and REM sleep. Indeed, a number of studies have demonstrated specific regional reactivations during post-learning sleep. For instance, a H2 15O PET study showed that several areas activated during procedural motor sequence learning were significantly more active during subsequent REM sleep [10]. The same lab also showed that hippocampal and parahippocampal gyrus previously recruited during a spatial memory task were reactivated during post-training SWS and, interestingly, the amount of this reactivation was positively correlated with overnight spatial navigation improvement [26]. Finally, an fMRI study demonstrated a significant reactivation in primary visual cortex during NREM sleep after intensive visual perceptual learning [27].
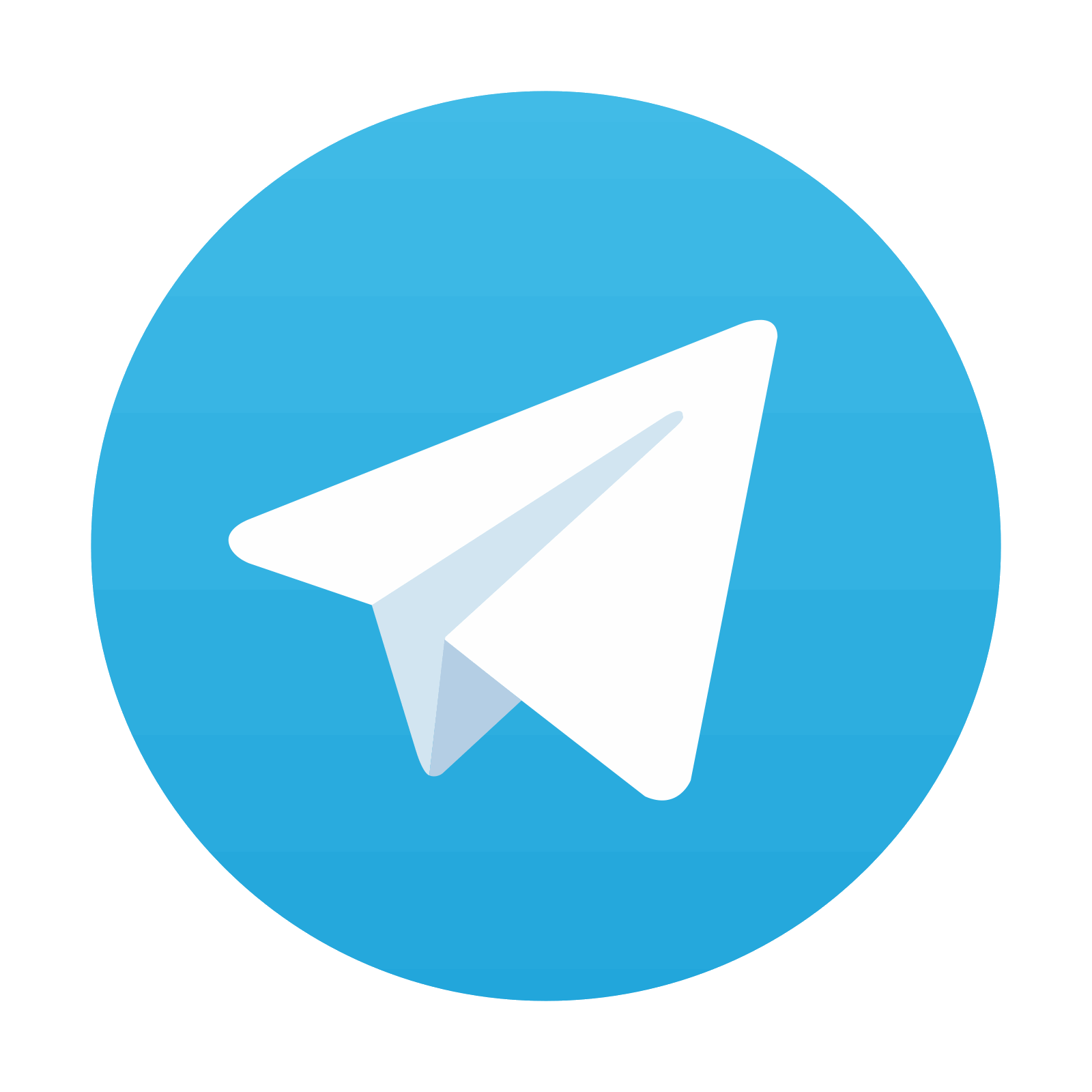
Stay updated, free articles. Join our Telegram channel
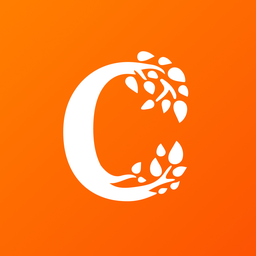
Full access? Get Clinical Tree
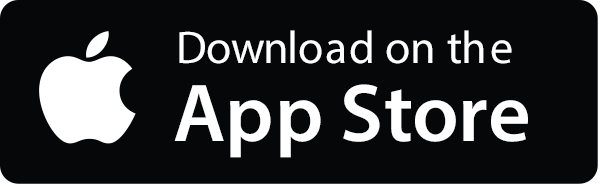
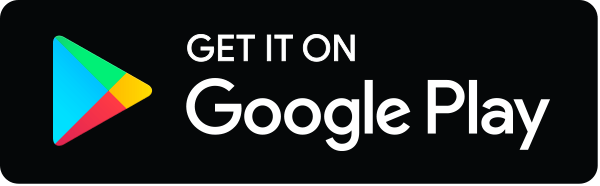