and Gordon N. Dutton2
(1)
Department of Psychology, LMU Munich, München, Germany
(2)
Department of Visual Science, Glasgow Caledonian University, Glasgow, UK
2.1 Visual and Oculomotor Functions and Their Significance for Visual Perception
The main foundations of the various perceptual capacities are built on peripheral (sense organs) and central components of the sensory systems, particularly in the cerebral cortex, which are specialised in the analysis and coding of sensory information, translating the outcome into experience, and making it accessible for use. Figure 2.1 shows a schematic sketch of the brain with the cortical structures relevant for vision, audition, body perception, language, and motor action. The integration of processed information and synthesis of the segregated outputs are essential prerequisites for coherent perception, experience and action (Goldstein 2010; Yantis 2013). A great deal of information about the environment is of a visual nature, i.e. the world within our brains is a predominantly visual one. The prominent role of the visual modality is, however, not only limited to perception. Major elements of experiences, memories and conceptions concerning the external world are based on vision, as are language (e.g. object labels), emotional experiences, and gross (e.g. walking) and fine motor actions (e.g. hand and finger movements). Finally, human curiosity, particularly in more distant space, i.e. beyond the space of grasp and touch, is mainly directed toward visual aspects of the surroundings. Children with cerebral visual disorders are therefore confronted with the very special condition of coping with a world that they can encompass only in part and with great effort, often without access to detailed prior visual experience and knowledge.


Fig. 2.1
Schematic sketches of the brain showing the regions specialised for various mental functions. (a) the left side of the brain. (b) the right side of the brain
According to the level of complexity, perceptual capacities can be classified as “lower” and “higher” visual capacities; another principle of classification refers to the type of visual information and material, which is processed. “Lower” or elementary visual capacities comprise the visual field, visual light and dark adaptation, visual acuity and spatial contrast sensitivity, colour vision, and spatial vision (localisation, distance and direction perception, monocular and binocular depth perception, including stereopsis). “Higher” or more complex visual capacities include visual identification and recognition, topological and geographical orientation, and text and number processing. Moment-to-moment visual mapping of the surrounding environment provides both the substrate for visual search and the principal means of bringing about guidance of movement through our surroundings (Goodale 2011). The various visual capacities are built on the availability and interplay of several visual functions, with varying degrees of involvement of cognition, which make them visual-cognitive abilities, but nevertheless represent genuine visual functions at different levels of organisation.
The visual system can be characterised as a sequence of structures and functions, which transform physical light stimuli into neuronal signals and process them at successively higher levels of organisation. At the most basic level, the visual system operates as an optical measuring system, comprising components that focus optical stimuli as images onto the retina of the left and right eyes with the help of the adaptation system, for mapping the image on corresponding retinal locations so that the two monocular images meld into one image with the help of the vergence system. Accommodation and vergence are coupled: in far vision, both lines of sight are in parallel, i.e. vergence is close to zero, while in near vision vergence increases as well as accommodation. The two retinal images are finally fused in the visual cortex to a coherent percept; the small disparity between the two retinal images is in addition used for finely graduated stereoscopic acuity and stereopsis.
The mobility of the eyes allows a more accurate inspection of objects and their details, respectively, by precise and accurate fixation. Mechanisms in the brain stem, the mid brain and the thalamus and in the cortex are involved in the guidance and control of eye movements, so that we can fixate stationary and moving visual stimuli (Fig. 2.2). Disorders of eye movements can therefore impair visual information processing and thus visual perception, either because oculomotor scanning and fixation shifts in space are impaired or because precise fixation of stimuli for further fine analysis becomes difficult if not impossible. The visual system and the oculomotor systems are intimately connected with each other, and have to co-operate closely to enable fast and reliable visual information processing.


Fig. 2.2
Schematic sketch of CNS structures involved in the guidance of saccadic eye movements. PFC pre-frontal cortex, FEF frontal eye fields, SEF supplementary eye field, PC posterior parietal cortex, V1 the visual cortex (Modified after Pierrot-Deseilligny et al. 1995)
Cognition, in particular, attention and executive functions, and motivation are also involved in visual perception and contribute to the reliability and efficiency of the visual system (see Chap. 3). The close temporal and spatial interplay of the visual system with cognitive, and eye and head movement systems, guarantee high speed, reliable visual information processing and coding even in complex visual task conditions in daily living activities. Table 2.1 shows essential prerequisites for the reliable representation of the visual world in the brain.
Table 2.1
Prerequisites for an accurate and reliable representation of the visual world in the brain
Adequate differential sensitivity for visual stimulus dimensions and characteristics (resolution, brightness, shades of grey, colour, hue, size, form, orientation, position, depth, direction, etc.); |
Selection of features for discrimination and identification (recognition) of complex stimuli (objects, faces, scenes, text information), including parallel and serial synthesis of single features to a whole; |
Coding and storing of visual stimuli and their characteristic features in visual memory that are relevant for further identification and recognition; |
Identification/recognition of complex visual stimuli despite differing conditions ´(visual constancy capacities) |
Association with additional important information (use, denomination, episodic context, past experience) |
Temporary storage of processed visual information until discrimination, identification, recognition and associations are complete (visual working memory) |
Long term storage of processed visual information (visual long-term memory) |
In summary, the various functions and capacities of visual perception can hardly be reliably separated from each other, either because they are based on each other (e.g. visual acuity on accommodation; detection and spatial localisation of stimuli on visual field extent; visual identification and recognition, on colour, form, size and shape processing), or they depend on each other (e.g. fixation of stimuli and spatial localisation; visual acuity and fixation accuracy, and vice versa). Any classification of visual functions and capacities is, therefore somehow artificial, but is helpful to denote and define the particular functions and capacities described, and to better explain and understand the interplay between them. Table 2.2 contains a compilation of the visual and oculomotor functions and capacities and their respective functional significance. “Function” refers to basic components of vision. They subserve but do not represent genuine visual-perceptual capacities. Such functions include, for example, oculomotor components, visual adaptation, and contrast sensitivity. “Visual capacities” refer to components of visual perception that support more complex visual abilities, (e.g. visual acuity for form vision, form vision for object and face perception).
Table 2.2
Summary of visual sensory and oculomotor functions and capacities and their significance in the context of visual perception
Visual field | Overview; detection and localisation of stimuli; parallel processing of spatially distributed stimuli (“simultaneous perception”); hazard detection; guidance of movement |
Spatial contrast sensitivity | Spatial resolution of contours and form elements; visual acuity, stereoacuity |
Visual acuity | Form vision, stereoacuity, text processing |
Colour vision | Object identification and recognition |
Form vision | Object, face, and scene perception; text processing |
Space perception | Localisation, distance and direction perception, monocular and binocular (stereopsis) depth perception; topographical orientation |
Object perception | Identification and recognition of objects of different categories |
Face perception | Identification and recognition of (familiar) faces, including age, gender and facial expression |
Accommodation | Contrast sensitivity, visual acuity, stereoacuity, form vision |
Vergence | Binocular vision, visual acuity, stereopsis |
Saccades | Transport of the fovea to targets; scanning of objects, scenes, and text material |
Smooth pursuit eye movements | Fixation of a moving target |
OKN | Stabilisation of visual perception by resetting the eyes during prolonged movement of the surrounding and Direct gaze toward oncoming visual stimuli |
VOR | Stabilisation of visual perception during body motion |
2.2 Neurobiological Foundations of Vision
The neurobiological foundation of visual perception is mainly in the retina and geniculo-striate pathway (optic tract, optic radiation), and the striate cortex, which is located in the posterior brain (occipital cortex). The visual cortex consists of the primary visual cortical area (striate cortex, Brodmann area [BA] 17, V1) and the so-called extrastriate or visual association cortex, which consists of more than 30 areas that differ with respect to the processing and coding properties of their neurons, and their connections with other visual and non-visual areas. These ‘higher-order’ visual areas process and code the various dimensions of visual information, e.g. brightness, contour, orientation, form, colour, motion, spatial attributes (position, distance, direction, etc.), and complex visual stimuli, e.g. objects, faces, and places and routes (landmarks) (Goldstein 2010; Yantis 2013; Zihl 2014). The central visual system – like other central sensory systems – shows parallel organisation with hierarchical components. This organisation principle is known as “functional segregation” or “functional specialisation” (e.g. Zeki 1993; Cowey 1994; Rainer and Logothetis 2003; Grill-Spector and Malach 2004), which increases over the course of development (Dobkins 2009). This model implies parallel and serial processing and coding in terms of division of labour, i.e. each area is more or less specialised for the processing and coding of a particular visual stimulus dimension; the aggregated organisation is based on parallel and serial processing modes. The various visual areas are reciprocally interconnected; exchange of information and thus interaction is possible in bottom-up (stimulus-driven) and top-down (intention-driven) directions. Within the extrastriate visual cortex, prominent processing pathways have been identified, which are either specialised in processing and coding spatial information (the occipital-parietal or WHERE-pathway) or object properties (the ventral, occipito-temporal, or WHAT pathway) (Desimone and Ungerleider 1989). This two-route model of visual spatial and visual object information processing has been converted into a two-route model of visual action (dorsal route) and visual perception (ventral route) (Goodale and Westwood 2004; Milner and Goodale 2006, 2008; Goodale and Milner 2010). Although there is some neuropsychological evidence for dissociation of either visual- spatial/visual object processing and visual-action/visual-perceptual deficits after injury to the ventral and dorsal route, respectively, both models may be too simplistic to explain visual perception as a coherent, integrated outcome of processing and coding (Schenk 2006; Schenk and McIntosh 2010; De Haan and Cowey 2011). In particular, the interplay between visual areas and both global and local integration of the various pieces of information into a coherent percept as a whole, and the various parallel and serial convergent and divergent processes within and between the two routes, which allow the processing of a large amount of information within a given time window and in the actual context of perception, intention and action, cannot be fully explained by either model. Co-operation and interaction between the two streams and other components of a distributed network involving also posterior and inferior parietal and prefrontal structures guarantee spatial and temporal coherence of processing of visual information via attention and working memory, also subserving visually guided action and spatial navigation (Goodale and Westwood 2004; Konen and Kastner 2008; Singh-Curry and Husain 2009; Kravits et al. 2011). The dual route model of cortical visual processing in terms of WHERE (dorsal route) and WHAT (ventral route) is still used in the literature and there is evidence, that in the context of focal brain pathology affecting these brain areas this model offers some sense and utility (e.g. Dutton and Jacobson 2001; Dutton 2009; Klaver et al. 2011). For the sake of simplicity, we too refer to this model in the context of describing behavioural manifestations of damage to the visual brain without implying that it exhaustively explains visual perceptual disorders.
Interestingly, early clinical and pathological anatomy observations are in support of the functional specialisation model of the visual brain because of selective loss of single visual capacities, for example, colour vision, visual space perception, and visual recognition (Zeki 1993; Kanwisher 2010; Barton 2011; Zihl 2011). In addition, the visual brain is able to identify and recognise visual stimuli despite changes in their appearance, and thus in the images produced on the retina, concerning, for example, brightness, colour, shape, orientation, location, and perspective of the observer. This capacity is called visual perceptual constancy, and is part of visual concept learning, which requires perceptual organisation and is based on bottom-up and top-down processes (Quinn and Bhatt 2001, 2009). It plays an important role in the adjustment of the visual system to the perception of an object or object property as constant even though our sensation changes (Goldstein 2010; Yantis 2013), and depends on occipito-temporal (ventral) structures (Turnbull et al. 1997; Goldstein 2010; Yantis 2013). For the sake of simplicity, we refer also to this model without implying that it too exhaustively explains visual perception.
Visual information is transmitted from the retina via the optic nerves, lateral geniculate bodies, optic tracts and optic radiations to the primary visual cortices (V1), from where it is distributed to areas in the visual association cortices (extrastriate visual areas) for further processing (Fig. 2.3). As already mentioned, these visual areas are specialised for the processing and coding of visual stimulus dimensions, for example, colour, form and shape, motion, and spatial aspects (location, distance, direction, and depth). Brain imaging studies in healthy subjects have confirmed the model of functional specialisation of the visual cortex, and have also added additional empirical evidence for the dual-route processing model, showing that processing of spatial information is associated with more dorsal activation, while processing of object properties, objects and faces, etc. is associated with more ventral activation (Corbetta et al. 1991, 1993; Tootell et al. 1996; Kravits et al. 2011; Sewards 2011). Disturbance of one of these areas causes selective loss of the respective visual capacity; thereby visual spatial impairments are typically associated with dorsal injury, while impairments in the perception of colour, shape, objects and faces are associated with ventral injury (Kanwisher 2010; Zihl 2011; see also Sect. 4.3).
The striate cortex, and extrastriate visual areas are topographically organised, i.e. they possess a representation of the visual field. This topography is very precise in V1, where the central 15° of visual field cover around 37 % of the striate cortex (Wong and Sharpe 1999), and is characterised by high topographical precision, such that a definite retinal position (central visual field) or area (peripheral visual field) corresponds to a definite cortical position or area in V1, that correlates with the diameter of the receptive fields of neurons (Daniel and Whitteridge 1961; Wandell et al. 2007; Benson et al. 2012). The fovea comprises 0.5° on either side of the centre of the visual field (total diameter: 1°), while the macula extends to a radius of 4.5° (total diameter: 9°). There is still no accepted definition on the diameter of the fovea, which according to various authors varies between 0.8 and 2° of visual angle. There is convincing evidence that the central 0.5° of the visual field is represented in both striate cortices; this explains foveal sparing even after total destruction of one striate cortex (Huber 1962; Bunt et al. 1977; Sharpe et al. 1979; Horton and Hoyd 1991). The topographical representations of the visual field in extrastriate visual areas become more and more imprecise, i.e. receptive fields become larger and larger, and eventually cover one quadrant or one hemifield, and may even extend into the other visual hemifield, whereby the magnification factor decreases with eccentricity but remains constant in extrastriate visual areas when related to striate cortex conditions (Dumoulin and Wandell 2008; Harvey and Dumoulin 2011). Top-down (executive, attentional, motivational and emotional) influences modulate selection of particular inputs to visual cortical neurons and enable them to “assume different functional states according to the task being executed”, in an adaptive way by means of feedback pathways (Gilbert and Li 2013, p. 350).
2.3 Development of Vision and of Its Neurobiological Foundations
To a major extent, vision is a skill that depends on learning and use, just as other skills such as walking or talking. The development of vision is characterised by a more or less regular sequence. Periods of morphological development are paralleled or followed by functional development, mostly influenced and guided by environmental factors, i.e. the disposition and nature of visual stimuli. While the auditory system already shows an advanced functional developmental stage at birth, the visual system primarily commences its functional development after birth. In the first months, a baby shows rapid development in nearly all visual functions and capacities, although visual acuity and visual contrast sensitivity at distance still need months and even years to fully develop (Granrud 1993; Slater 1998a, b; Atkinson 2000; Braddick and Atkinson 2011). Table 2.3 summarises the normal development of visual and oculomotor functions and capacities in the first year.
Table 2.3
Development of visual and oculomotor functions and capacities
Age | Functions/capacities |
---|---|
Birth | Blinks (rapid closing and opening of the eye lids) |
Slow pupil responses to day light | |
Very limited accommodation | |
Visual acuity ~ 20/150 | |
Responses to light, motion, and colour | |
Oculomotor scanning of the visual environment in ~ 5–10 % of waking time | |
Oculomotor scanning and searching movements for e.g. Light stimuli or contours within a radius of about 45° | |
M 1–2 | Threshold for light detection decreases, visual acuity increases |
Oculomotor scanning and searching eye movements within a radius of 60–90° | |
Increase in precision of smooth pursuit eye movements | |
Incipient binocular vision starting at about 6 weeks | |
M 2–4 | Increase in accommodation power |
Conjugate eye movements in all directions | |
Eyelids close when stimuli (e.g. hand movements) appear suddenly before the eyes | |
Oculomotor scanning of the visual environment in ~ 30–40 % of waking time | |
Oculomotor scanning and searching eye movements within a radius of 180° | |
Baby observes his own hand(s) when manipulating objects | |
Baby shows defense reactions for objects approaching in collision course | |
M 4–6 | Visual acuity increases further |
Binocular vision is established | |
Visual identification/recognition of particular objects and faces/persons possible | |
M 6–12 | Visual acuity is 20/100 |
Baby avoids visual depth |
The development of the visual system also depends on the stage of maturation of the eye, and takes place in serial steps (Banks and Shannon 1993; Candy 2006; Iliescu and Dannemiller 2008). The critical period of development of the eye and particularly the retina occurs between the second and fourth month of pregnancy. The differentiation of the cellular retinal elements starts in the central retina, the fovea; the ganglion cells develop before the receptors. In the sixth month the eye lids open for the first time and the macula, the inner part of the retina (diameter: 10°) begins to differentiate; this process is completed several months after birth. At the time of birth particularly the receptors in the fovea are wide, have not reached their final density, and need visual stimuli for their functional refinement (Tian and Copenhagen 2003). This fact explains the poor visual acuity in this period of early childhood, which is about 10–30 times lower than that of an adult. At the end of the first year the development of the retina is nearly complete; however, smaller morphological changes can still be observed till the end of the fourth year. It is important to note that the development of visual spatial (and temporal) resolution of the visual system is tightly coupled with the development of the oculomotor system, in particular pupil movement, accommodation, and vergence (Schor 1985; Charman and Voisin 1993; Charman 2004).
The brain structures involved in visual information processing begin their development by weeks 13–15 of gestation (Chi et al. 1977; Table 2.4). The visual midbrain (lateral geniculate body, LGB) and the primary visual cortex (striate cortex, V1) develop rapidly; the thickness of the striate cortex and the number of interneurons and fibre connections within and between areas in the visual cortex, and between these areas and other structures of the brain increase. Visual modularity also increases over the course of development (Dobkins 2009), but development of visual cortical areas depends on stimulus-specific visual experience (Quin et al. 2013). The two main visual information processing pathways in the brain show different developmental timing. The ventral (i.e. occipito-temporal) visual pathway shows prolonged development and appear less “plastic” compared to the dorsal (i.e. occipito-parietal) visual pathway; in contrast, fibre connections within the ventral pathway mature earlier (Klaver et al. 2011; Dormal et al. 2012). The morphological development of the visual brain is the crucial basis for the functional development of the various substructures, and their co-operativity, as well as their connectivity with other functional systems, for example the attention, memory, executive and motivation and reward systems. Learning by selection is a fundamental principle in visual development; it depends on an efficient attentional mechanism for information processing (Amso and Johnson 2006). Attention also plays an important role in recognition memory development during early childhood (Rose et al. 2001); it modulates active vision in (spatial) selection and processing, but also in coding and representation of visual information (Berman and Colby 2009). Emotional evaluation of visual stimuli supports vision by enhancing selection of stimuli, based on the affective impact of past visual sensation and experience (Barrett and Bar 2009). ‘Affective learning’, i.e. repeated systematic experience with task-relevant affective information, for example, colours or faces, not only modulates visual association cortical areas and connected prefrontal structures, but also enhances response activity and functional connectivity within the primary visual cortex (Damaraju et al. 2009) indicating that stimulus-emotion associations are powerful learning conditions even at low-level visual processing stages. Interestingly, facial expression (“emotional faces”) in particular captures attention, i.e. children preferentially direct spatial attention in the direction of such faces (Elam et al. 2010).
Tables 2.4
Temporal development of regional brain structures
Lobe | Gestational age (weeks) | Sulci/fissures | Gyri |
---|---|---|---|
Occipital | 10–27 | Calcarine fissure; parieto-occipital and lateral; occipital sulci | Superior and inferior occipital gyri; lingual gyrus cuneus; occipito-temporal gyrus |
Parietal | 10–26 | Parieto-occipital fissure; interparietal sulcus; interhemispheric fissure; Rolandic and postrolandic sulci | Superior, middle, inferior parietal lobules; angular and supramarginal gyrus; cingulate and postrolandic gyri |
Temporal | 14–30 | Sylvian fissure; superior, middle and inferior temporal sulci | Superior, middle, inferior temporal gyrus; fusiform gyrus; parahippocampal gyrus |
Frontal | 10–28 | Superior and inferior frontal sulcus; cingulate sulcus; olfactory sulcus | Superior, middle, anterior gyri; insula; cingulate gyrus |
It appears that the prenatal development of the visual system occurs at a basic level without visual stimulation and associated experience. In contrast, the postnatal development of the visual system is both, environment- and practice-dependent; it is environment and action – “anticipatory”. Early visual experience is required for further development of the structures and functions of the visual system to make use of the visual information provided by the environment, and to develop the necessary adjustments to this visual environment (Berardi et al. 2000; Maurer et al. 2008; Lewis and Maurer 2009). This principle applies also to the development of the so-called receptive fields, i.e. the region of the visual field that is covered by a neuron, in which a stimulus can elicit the best response of this neuron, regarding its size as well as its specialisation of processing, and coding properties, and its interactions. Monocular visual deprivation, for example, caused by improper alignment of the eyes (known as strabismus, squint, or heterotropia), or anisometropia (a greater refractive error developing in one eye), causing amblyopia is associated with underdevelopment of the corresponding visual cortex: the number of functionally intact neurons decreases, the receptive fields do not fully develop or can shrink, and neurons do not arrive at the normally occurring high degree of specialisation in processing and coding of the finer details of visual patterns (Bi et al. 2011). Similarly, bilateral amblyopia can compound untreated bilateral refractive error (Abrahamsson et al. 1990) and developmental disorders affecting the peripheral part of the visual system, where the associated sensory deprivation can cause amblyopia, therefore lead to delayed or even impaired morphological and functional development of the central components of the visual system (Kiorpes and McKee, 1999; Sireteanu 2000; Maurer et al. 2005, 2008; Lewis and Maurer 2009). This delay or impairment may affect both the differentiation of the visual system as well as the specialisation of the neurons with regard to their processing and coding properties. Consequently, incoming sensory information may be processed only incompletely, imprecisely or incorrectly, and integration of information may be impaired, despite the central structures of the visual system being intact. This condition of additional bilateral amblyopia may thus induce or compound difficulties with the development of a sufficiently valid and reliable representation of the visual world in terms of fine differentiation within visual categories, the identification and recognition of visual stimuli, the guidance and control of motor activities and of complex actions, and the development and optimisation by perceptual learning.
2.3.1 Visual Field
Definition. The visual field is the extent of visual space over which vision is possible with the eyes held in a particular position, typically straight ahead. In the adult, the measurable binocular visual field extends over 70° of visual angle to the left and right side from the fovea, the upper visual field over 50°, and the lower visual field over 60°. Therefore, the total binocular visual field size is at least 140° in the horizontal and 110° in the vertical dimension (Zihl 2011). On confrontation testing the visual field can extend up to 100° temporally for each eye for detection of movement, and movement of one’s own foot can be perceived within a normal stride while looking straight ahead.
Newborn babies only respond to large, high-contrast stimuli that appear in their line of sight or close by, i.e. in the central portion of their visual field. Thus newborn babies are not blind for stimuli appearing in the visual field periphery, but do not respond because the extent of their visual field does not exceed ~ 30° (Maurer et al. 2008). However, even within the first month the diameter of the visual field extends markedly; at the age of 4–8 weeks the minimum size of the visual field is about 30° in both hemifields (Schwartz et al. 1987; Werth 2007, 2008). The quantitative assessment of the visual field classically requires accurate and stable fixation of a central stimulus and a valid response to the appearance of a peripheral stimulus as indicator for its detection (see Chap. 6). Both requisites do not reliably exist in the first months. However, searching eye movements can be observed within a radius of 60–90° by the end of the second month (the so-called field of search). At the end of month 4, the field of search expands to a radius of about 180°, i.e. 90° to either side. This observation may be taken as evidence that at the end of the fourth month the visual field allows a sufficient view over the visual surroundings, provided that the stimuli are large, of high-contrast, and/or are moving. An essential prerequisite for detecting peripheral stimuli and being responsive in terms of orienting responses (eye and head movements, grasping) is an adequate level of alertness and attention, and of visual curiosity for the visual world. These prerequisites are not always sufficiently available in the first months (Richards and Hunter 1998). At the beginning of the second year, children show a visual field extent similar to that of older children and even adults when kinetic perimetry is used (Dobson et al. 1998; Delaney et al. 2005; Werth 2008). From the age of 2 years on, kinetic perimetry can be used for the assessment of the visual field (Cummings et al. 1988; see Fig. 2.4), while automated eye movement detection methods now can be used to objectively test attentional visual fields in young children (Murray et al. 2013). It has to be considered, however, that at this age the field of attention has still not fully developed, and young children may, therefore, ignore peripheral stimuli, particularly when they appear simultaneously in both hemifields.
2.3.2 Visual Adaptation
Definition. Visual adaptation allows the flexible adjustment of vision to changing light intensities in the mesopic (daylight conditions), photopic (higher illumination levels), and scotopic conditions (night conditions). Thus, visual adaptation is the ability of the visual system to adjust to higher levels (light adaptation) or to lower levels of light (dark adaptation), in order to restore visual sensitivity (acuity, contrast sensitivity). Light adaptation takes a few minutes, while complete dark adaptation takes up to 30 min (Rushton 1972).
Thresholds for detection of light stimuli are increased for all three conditions in the first weeks, but decline to about the adult levels at the end of the third month (Brown 1990). Thus in the first 3 months infants are only minimally protected, particularly from intense light levels, and are therefore very sensitive to bright light, which causes discomfort from glare. In contrast, time for dark adaptation in children may be similar to adults (Hansen and Fulton 1986; Fulton and Hansen 1987).
2.3.3 Visual Contrast Sensitivity and Visual Acuity
Definition. Visual contrast sensitivity (spatial contrast vision) refers to visual spatial resolution, and defines an individual’s capacity to detect subtle differences in light and dark shading in a pattern, an object, or a scene. In the adult, visual contrast sensitivity is highest for gratings of intermediate spatial frequencies (4–8 cycles/degree) and lower for both coarser and finer gratings. Visual acuity is the individual’s capacity to detect small spatial gaps in a figure (e.g. circle or square) of maximum contrast (black and white), or between two parts of a figure (minimum separabile), to discriminate and identify single forms, letters, or numbers, or to process text material (reading acuity) (Zihl 2011). Formal measures specify the lighting conditions.
The capacity to discriminate visual stimuli with high accuracy and reliability depends crucially on the spatial resolution power of the visual system. Visual spatial resolution is the basis of visual spatial contrast sensitivity, and represents also an essential prerequisite for visual acuity and stereopsis. In comparison with adults, spatial contrast sensitivity in neonates is lower by a factor of 50, while visual acuity is lower by a factor of 40 (Banks and Shannon 1993; Maurer and Lewis 2001a, b; Cioni et al. 2006). In children, visual spatial contrast sensitivity is assessed behaviourally using preferential looking paradigms (PL) and registration of orienting eye movement responses. Both methods make use of the fact that children show preferred orienting responses to gratings that they can see as patterns, i.e., they can discriminate gratings differing in spatial separation (width of bars) and contrast (contrast between bars). Another method is to record pattern-evoked visual potentials; the amplitude of P1 can be taken as an indicator for contrast sensitivity (for details, see Chap. 6). Figure 2.5 shows the results of oculomotor orienting responses, preferential looking, and visual evoked potentials to gratings differing in spatial frequency and contrast sensitivity for months 1, 2, 3 and 4 (Hainline 1998). There is a striking increase in sensitivity in this developmental period, with respect to both spatial frequency and contrast; in addition, the peak of sensitivity moves towards higher frequencies. While the development of sensitivity for lower spatial frequencies appears more or less complete at the end of year 4, sensitivity for higher spatial frequencies (>10 cycles/degree, c/deg) appears complete by the age of about 8 years (Gwiazda et al. 1997; Ellemberg et al. 1999; Maurer and Lewis 2001a, b; Adams and Courage 2002; Cioni et al. 2006; Daw 2006). The gradual increase in spatial contrast sensitivity (and also of visual acuity) can be explained by the concomitant morphological development of the fovea (Cioni et al. 2006; Daw 2006).


Fig. 2.5
Development of contrast sensitivity between the 1st (a) and the 4th month (d) of life depending on the method of assessment (Adapted from Hainline 1998). © Psychology Press reprinted with permission). ●–●: saccadic orienting responses;
: forced preferential looking; ▾– –▾ visual evoked responses (VEP). Note that for VEP-responses higher contrast sensitivity values were obtained compared with behavioural responses

Concerning near vision in young children, contrast sensitivity may be sufficiently developed for adequate discrimination and recognition of complex visual stimuli (objects, faces) and stimulus details by the end of the first year. Valid and reliable assessment of visual acuity is possible during infancy (Mash and Dobson 2005), but is more accurate by about the second year (Lithander 1997). Table 2.5 shows visual acuity values for form, assessed in 89 children aged 2–4 years (Lithander 1997) Spatial resolution for form (so-called minimum separable) increases considerably in this period from 2.10 to 1.30 min of arc, while Snellen acuity increases from 6/12 (0.48 decimal; 0.3 logMAR) to 6/8 (0.77 decimal; 0.1 logMAR). Thus visual acuity increases by about fivefold in the first 6 months, and by the age of 6–7 years visual acuity appears more or less fully developed (Maurer and Lewis 2001a, b; Duckman 2006).
Table 2.5
Minimum separable (MS; in min of arc) and Snellen-values for 89 children between 2 and 4 years of age
Age (months) | MS (SD) | Snellen |
---|---|---|
24–29 | 2.10 (0.60) | 0.48 |
30–35 | 1.81 (0.41) | 0.55 |
36–41 | 1.49 (0.26) | 0.67 |
42–48 | 1.30 (0.24) | 0.77 |
2.3.4 Colour Vision
Definition. Colour vision is the capacity to distinguish visual stimuli on the basis of the wavelengths of light they emit. The discrimination of colour hues depends on the functional capacity of the cones, which has developed incompletely by the end of the first year (Banks and Shannon 1993). However, even newborns show evidence of a kind of rudimentary colour vision (Abramov and Gordon 2006); by the age of 4 weeks children can discriminate green and red (Adams and Courage 1995). The rapid development of light and contrast sensitivity in the first 2 months is followed by the development of sensitivity to colours, provided that the colour stimuli are large and of high contrast (Brown 1990; Abramov and Gordon 2006). Table 2.6 shows the development of colour discrimination for primary colours; discrimination of red, green and blue may be detectable even earlier, if coloured OKN stimuli are used (Zemach and Teller 2007; Zemach et al. 2007). Coloured stimuli possibly appear less intense during the first months, because children at this age require a higher colour contrast for accurate discrimination (Morrone et al. 1990; Allen et al. 1993; Teller and Lindsey 1993). However, children may be able to use colour as a feature to discriminate, identify and recognise objects. If they are confronted with coloured and colourless objects, they prefer the coloured ones; thus colour represents an already prominent visual attribute in early childhood (Catherwood et al. 1996). At the age of 6 months, the capacity for colour discrimination appears similar to adults (Franklin and Davies 2004; Abramov and Gordon 2006).
Table 2.6
Development of colour discrimination under isoluminant conditions
Age (weeks) | Discriminable colour hues |
---|---|
4 | Red-green |
8 | Red-green, red-blue, red-purple, red-yellow, green-yellow |
12 | Red-green, red-yellow |
2.3.5 Visual Space Perception
Definition. Visual spatial functions comprise the processing of spatial properties of optical stimuli and include position, distance, depth, and direction (horizontal, vertical and straight ahead) as well as of spatial relations of stimuli (objects) in a scene, of both a three- and a two-dimensional nature (Zihl 2011).
Even during the first days after birth, babies can locate a visual stimulus within a radius covering 45°, but localisation accuracy is low (Roucoux et al. 1983). At the age of 5 months babies can execute accurate saccadic eye shifts to stimuli (Hainline 1998), while saccadic accuracy corresponds to that of adults by the age of 4 years (Fukushima et al. 2000). In early childhood, the perception of distance in the straight-ahead direction is better in the binocular than in the monocular viewing condition. By the age of 5 months babies can reach correctly for visual objects under monocular conditions in 65 % of stimulus presentations, but in 89 % of trials under binocular conditions (Granrud et al. 1984). Before this age, babies do not show systematically guided reaching actions, although distance perception may be present even earlier (Daw 2006; Stiles et al. 2008). More complex visual-spatial capacities, including representation and translation of visual spatial information into visually guided control when copying or drawing, require many years of experience and practice and are not sufficiently developed before the age of 9 years, whereby perceptual abilities precede space representation and graphomotor abilities (Del Guidice et al. 2000).
Stereopsis develops rapidly between months 3 and 6; stereoacuity improves within a few weeks from very low levels of acuity to disparities of ~1 min of arc (Held 1993). At the end of the first year stereoacuity increases further to 30–40 s of arc, provided that contrast sensitivity and vergence are appropriately developed. Stereoacuity is eventually fully developed at the age of 6 years, with girls showing significantly better stereoacuity values between 4 and 6 years (Hainline 1998; Daw 2006; Duckman and Du 2006; Pola 2006).
2.3.6 Form and Object Perception
Definition. Form and pattern perception comprises the discrimination, identification and recognition of forms and Gestalten on the basis of their spatial features, e.g. length and orientation of lines, and spatial pattern properties. Object perception refers to the same visual capacities concerning real objects, which are characterised by global (e.g. size, shape) and local (e.g. form, figural and colour details) properties, and by special (shared only by a single object or object class), and generic features (shared by all objects of a category, but also other objects, not belonging to that category).
Both, simple and complex visual stimuli are available for the newborn baby in its “new” world, but at this time the visual system is hardly able to process and code such information. Alertness and curiosity are two fundamental prerequisites for early visual activities of the baby (Table 2.7). Despite the limited stage of development of the peripheral and central visual systems at this age, babies show remarkable preferences for more complex visual stimuli (see Table 2.8). This incongruity between the physiological stages of development and the perceptual preferences exhibited has been interpreted in favour of inherent visual capacities (Maurer et al. 2008; McKone et al. 2009; Hunnius and Bekkering 2010). In particular, faces attract attention, and are fixated for longer than other complex visual stimuli (Elam et al. 2010; see below). The combination of visual curiosity, indicating motivation for information supply, focusing and maintenance of attention and fixation upon faces, and repeated processing of faces, appears particularly attractive for the rapidly developing visual brain, suggestive of specific stimulus preference and prior expectation, respectively. Face processing requires close coordination of and cooperation between engaged visual cortical areas, but also cognitive and motivation/reward systems, and thus facilitates and promotes the bottom-up and top-down interplay of the functional systems involved.
Table 2.7
Some fundamental associations between context conditions and early visual perceptual activities
If the baby is awake and alert and light is not too bright, eyes are open |
If light conditions are appropriate, but no forms/patterns are in the line of sight, the baby begins to search for contours with the help of gaze shifts |
After a contour has been found, search stops, and fixation remains at the contour or close to this for a longer time |
Table 2.8
Preferred (italics) and less attractive visual stimuli for infants
High contrast vs. low contrast stimuli |
Larger vs. smaller forms and objects |
Moving vs. stationary objects |
Patterned vs. plain stimuli |
Horizontal vs. vertical and oblique lines |
Curvilinear vs. rectilinear patterns |
3–dimensional vs. 2-dimensional objects |
Objects in direct line of sight vs. peripheral |
Faces and face–like stimuli vs. other forms |
As mentioned above, newborns do not find all kinds of visual stimuli equally attractive and interesting, as indicated by oculomotor orienting responses and fixation times. At the age of 6 weeks babies can discriminate orientations of contours. Given the developmental state of contrast vision and visual acuity, it is not surprising that they prefer objects with coarse contours and lines in contrast to fine form or figural details. At the age of 3 months children can discriminate angles built from contours. At 4 months of age they can use form and object features for identification and recognition; the integration of features to a whole (‘Gestalt’) is, however, not possible before month 10. The development of form and pattern perception is characterised by an early tendency for the construction of Gestalten. Consequently, children show a stronger bias in terms of faster responses towards global processing of complex forms, at least up to the age of 10 years (Mondloch et al. 2003). With increasing visual development, and thus an increase in visual perceptual experiences, in cognitive capacities (attention, memory, executive functions) in general, and specific capacities involved in visual perception, young children prefer increasingly complex visual stimuli (Aslin and Smith 1988; Johnson 2003; Sireteanu et al. 2003; Maurer et al. 2008). Visual selection processes (Amso and Johnson 2006) and memory for complex visual stimuli (Rose et al. 2001) play an important role in the development of object perception in the first year of life. Viewing preference during visual and manual exploration grows rapidly during the first 3 years of age; in this period children prefer planar views and views of objects in an upright position in preference to other viewing directions and spatial axes (Pereira et al. 2010). Development of visual object recognition shows fast progress between 6 and 11 years of life, indicating that this complex ‘higher-order’ visual capacity requires intensive visual experience, with integration of the various processes involved, including figure-ground separation, form and figure completion, segregation of overlapping figures, and object constancy (Bova et al. 2007).
2.3.7 Visual Categorisation, Concept Formation and Constancy
The visual world offers ecological structures and spatial stimulus organisation, which facilitate and support the coherent perception of objects, scenes, humans and events (Gibson 1979). It appears that infants possess, at an early stage, various perceptual organisation principles that serve as a means of arranging the visual world into perceptual categories. Concept formation allows the child to identify and recognise figures, forms and objects, independent of external factors, e.g. lighting, shadows and occlusion of parts, visual context etc., as well as of internal factors related to the observer, e.g. perspective, distance, and episodic context. Concept formation is the basis for visual constancy, i.e. an object keeps its identity despite changes in internal or external factors. Constancy guarantees increasing independence of (concretistic) visual perception from the actual appearance of a visual stimulus, and thus enhances autonomy for action. The final product of concept formation is the ‘prototypical’ Gestalt, without losing the progressively developing capacity for fine discrimination and differentiation of stimuli and stimulus features within a given category. Elementary form and object constancy can be observed soon after birth indicating that constancy is most likely an innate capacity. Size constancy can be demonstrated around month 7 (Aslin and Smith 1988; Slater et al. 1990; Johnson 2003; Rakinson and Oakes 2003; Sireteanu et al. 2003; Maurer et al. 2008). Table 2.9 shows the typical sequence of Gestalt constancy principles in development. Gestalt principles facilitate the discriminability and classification of visual objects, and promote thereby the further development of object constancy, but top-down influences guiding perceptual learning in terms of organisation and categorisation of visual information and visual concept formation, and their interactions with bottom-up processes are crucial for gaining valid and reliable constructs about the various aspects of the visual world (Quinn and Bhatt 2009).
Table 2.9
Temporal sequence of the appearance of Gestalt principles in visual development
Principle of similarity: as of M 3 |
Principle of closure of forms and figures: as of M 3–4 |
Principle of coherence of objects and parts of objects: as of M 3–5 |
Principle of object segregation and object abstraction (figure-ground, continuity, effective Gestalt): as of M 8–10 |
The combination of the faculty of discrimination of complex visual stimuli on the one hand, and the accuracy and reliability of their classification and categorisation, respectively, on the other, is a good example of the close matching of apparently opposite developmental principles of the visual brain. The marked increase in visual information processing capacity does not develop without concomitant development of rules concerning how visual information has to be allocated and classified for saving of processed and classified information in visual memory. Part of this process is the definitely challenging ability to assign specific stimulus features, e.g. colour, form and size, to different objects, i.e. to be able to discover that different objects, e.g. ball, apple, bowl, share common features (form, colour, size), irrespective of the fact that they belong to different object classes. Repeated systematic practice with the identification and recognition of different objects sharing common features or properties, results in reliable and fast coding and representation of objects in visual memory. This representation also comprises knowledge about variations in appearance, semantic and pragmatic significance, use, and eventually categorisation and denomination. This object information constitutes the basis for the formation of modality-specific, i.e. visual, and supra-modal concepts of the objects in question (Quinn 1998, 2003; Quinn and Bhatt 2001; Rakinson and Oakes 2003). Although infants as young as 3 months are able to use various visual Gestalt cues to organise visual patterns, e.g. common motion, connectedness, continuity, proximity (Quinn and Bhatt 2009), it is not surprising, that top-down visual organisation processes in scene and object recognition, and the underlying processes in the brain, need several years of development, because intensive and repeated perceptual and concept learning is required, which is probably not complete until the age of 11 years (Bova et al. 2007).
Categorisation requires concept formation. Even very young children can recognise an object or face seen under different conditions, especially when these conditions possess high ecological relevance for the child. The ability to form concepts can be understood as an essential prerequisite to attain increasing familiarity with perceptual effects, and thus progressive certainty and safety in manipulating objects. Elementary visual memory capacities can be observed by a few weeks after birth, with rapid improvement over the first 12 months (see Sect. 2.3.8). The development of visual memory is possibly enhanced by the innate visual stimulus preferences (see Sect. 2.3.6). A special case of visual stimulus preference is faces.
2.3.8 Face Perception
Face perception represents a special case of object perception, which refers only to facial properties and attributes, both within and between facial categories. Visual face perception plays a particular role within visual development. Babies already prefer faces shortly after birth, i.e. without prior perceptual experience; this observation has led to the assumption of an innate perceptual mechanism underlying the preference of faces and facial expressions (Pascalis and Slater 2003; de Haan 2008; McKone et al. 2009). In the first weeks after birth, fast perceptual learning takes place, which is mainly devoted to faces, particularly the face of the mother or of another relevant person. This face, which is seen and thus processed with high daily frequency in different contexts, is favoured over other faces and “serves” probably also as a tool for the optimisation of visual social perception and acquisition of knowledge concerning the significance of facial expressions (see Sect. 2.3.10). This systematic and regular experience with one face, or a few familiar faces, is the basis for perceptual “tuning” in terms of progressive specialisation of the face-processing system (Simion et al. 2007) and the recognition and interpretation of facial expressions of other persons later in life (Mondloch et al. 2003; Bushnell 2011). Of course, the voice of the mother or of another relevant person also plays an important role (Sai 2005). At the age of 4 months children can recognise familiar faces even when typical, for example by a haircut (Turati et al. 2008). This implies that children at this age features are removed, can use characteristic individual facial features to identify a person. At the age of 6 months children prefer natural (‘real’) faces to photographs or line drawings of faces, independently of age, gender and skin colour of the faces (Rubinstein et al. 1999). As for objects, the development of the faculty of face discrimination is paralleled by an improvement in concept formation and consequently in face categorisation including face class (girls vs. boys, younger vs. older persons) and identity (father, siblings, grandparents, aunts, uncles, etc.). At the age of about 10 years, children’s representation of identity becomes expression-independent like in adults (Mian and Mondloch 2012).
Faces possibly also exhibit a high natural preference bias and accelerate visual learning, because their discrimination, identification and recognition, respectively, play a crucial role in social perception (see Sect. 2.3.10). Already in the first month children prefer faces to objects; at month 3 children fixate faces longer than objects, particularly the eye and mouth regions (Table 2.10). The identification and interpretation, (understanding) of facial expression probably develop in the second year; faces that do not mimic movements stop a child from smiling or trigger looking the other way. At the age of 3 months, children respond differentially to facial expressions: “happy” faces trigger positive responses; “sad” faces trigger negative responses (Table 2.11). This period is followed by a phase of imitation of facial expressions made by the mother (or relevant person). Eye contact plays a particular role in the perception and interpretation of facial expression. At the age of 2 months children show a vivid interest in eyes; at the age of 4 months they can discriminate between different directions of gaze of the other party. Reciprocal looking activates increasingly more selective attention (“joint attention”) and social responses in both communicating partners (De Haan and Nelson 1998; Pascalis and Slater 2003; de Haan 2008; McKone et al. 2009; Elam et al. 2010; see Sect. 2.3.10).
Table 2.10
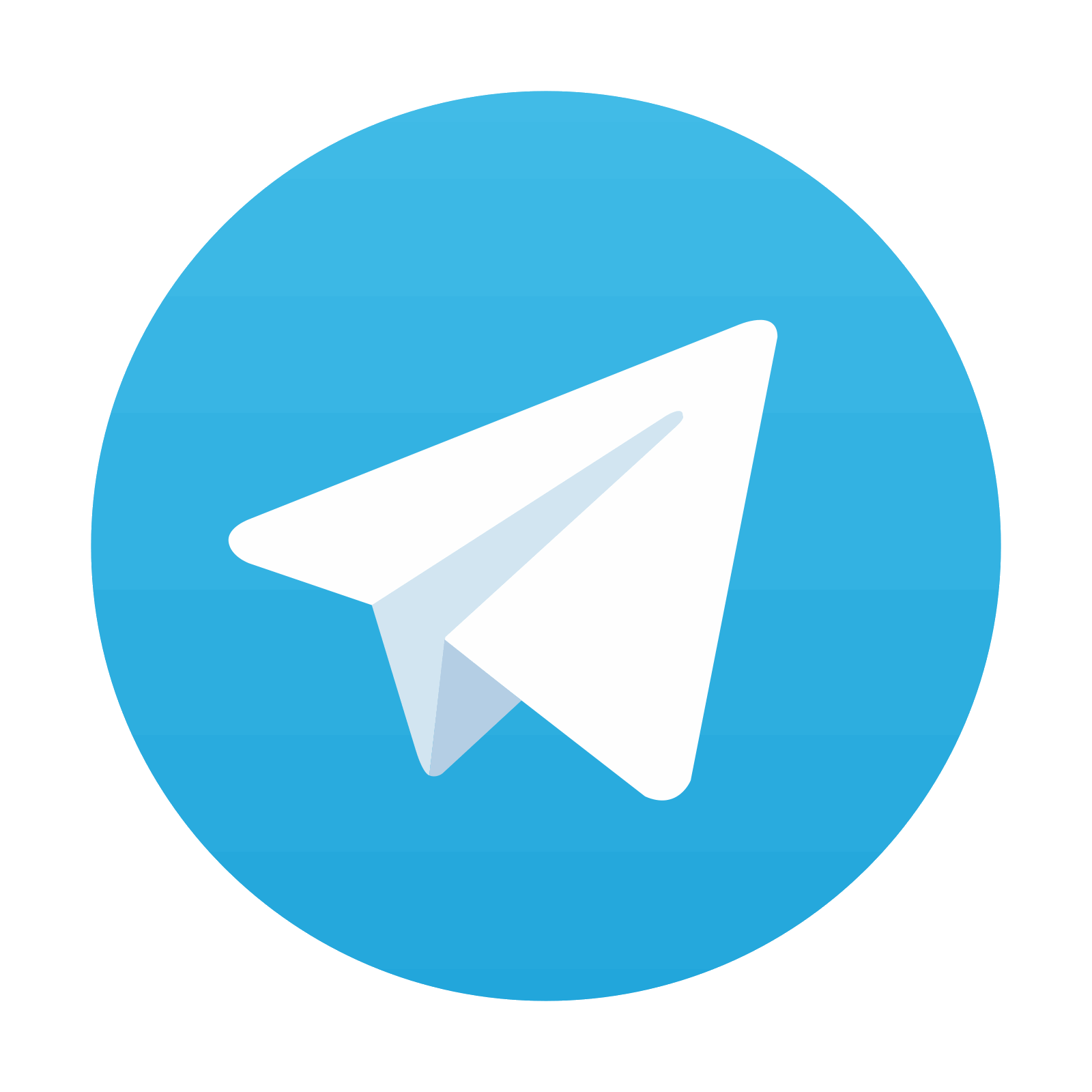
Inspection times (in % of total observation times) and smiling times (in % of inspection times) of 3–6 month old children for different face variations and for an object with similar figural complexity
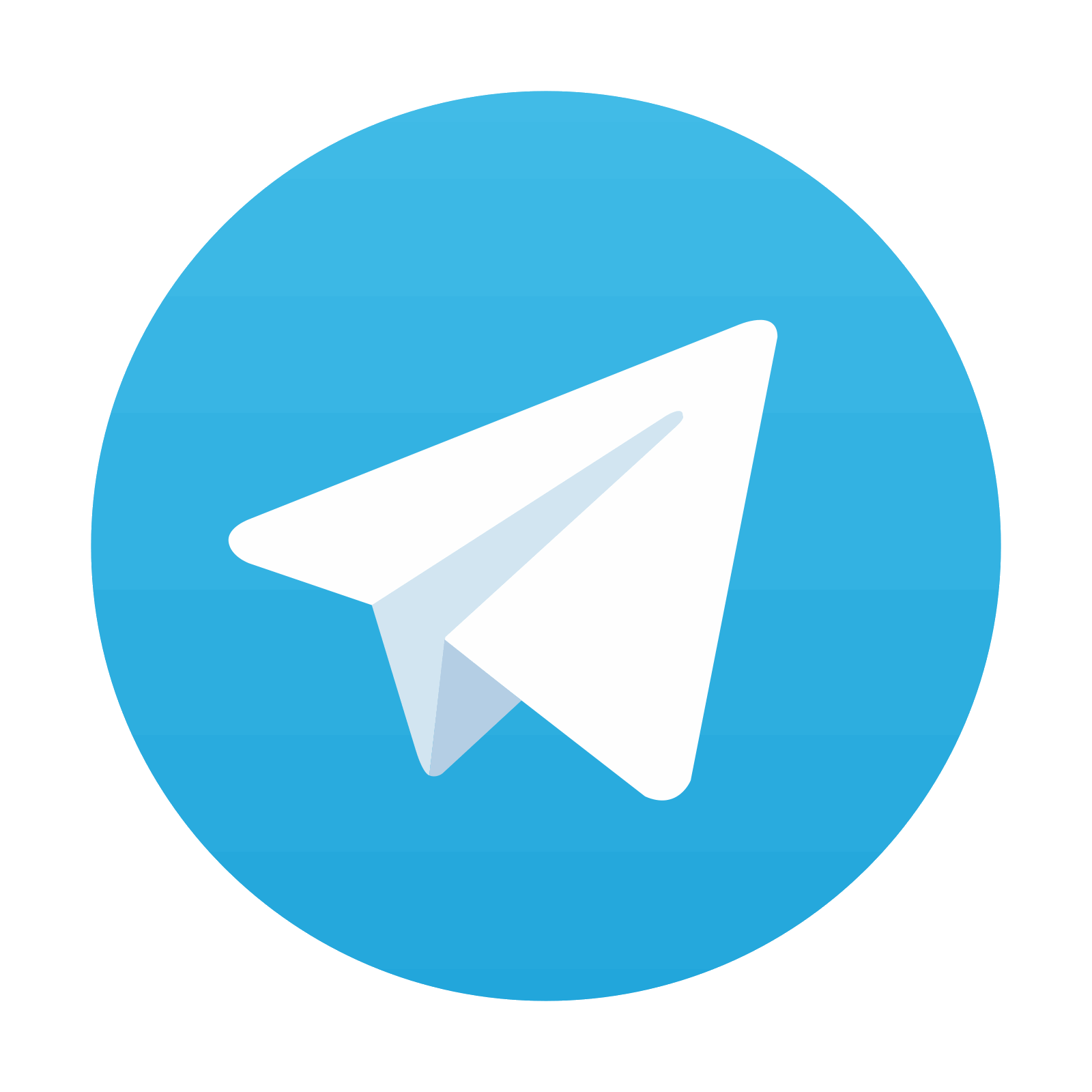
Stay updated, free articles. Join our Telegram channel
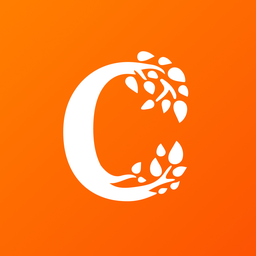
Full access? Get Clinical Tree
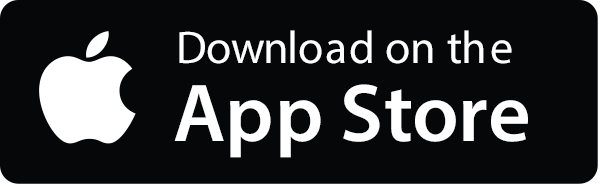
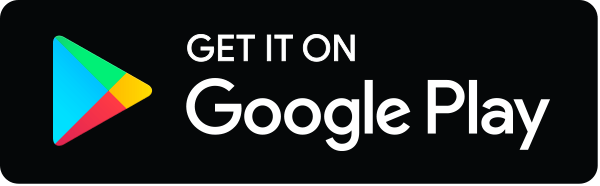
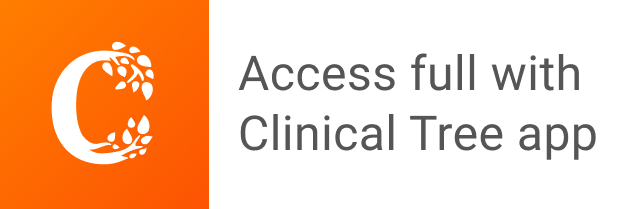