5 Differences between Human and Animal Discs: Pros and Cons of Current Animal Models for Preclinical Development of Biological Therapies for Low Back Pain
Jeffrey C. Lotz
Abstract
Animal models are an important component of preclinical therapy development for disc degeneration and discogenic pain. These models demonstrate the biological response and functional performance within context of in situ stressors that include load, host cells, and transport. Unfortunately, there is no consensus on the preferred model for preclinical purposes. Animal model factors that are commonly debated include disc size, cellular content, tissue composition, and in vivo loading. This review summarizes current literature regarding these factors and discusses how they should be integrated into the decision making for those developing biological therapies for the disc. Ultimately, the most significant barrier for identifying the ideal model is ambiguity regarding disc pain mechanisms in humans, and the lack of validated pain measures in animals that forecast clinical trial outcomes.
Keywords: animal models, low back pain, spinal disc degeneration
5.1 Introduction
Animal models allow investigators to collect data on physiological and pathological conditions that form the basis for developing clinical interventions. These models also provide a critical therapy development tool, and form a central component of preclinical evaluation of biological treatments for disc degeneration–related back pain. In situ factors such as physical stress, transport, immunity, and tissue spatial relationships will undoubtedly influence the retention and activity of regenerative products. Equally important, the consequences of therapy activity such as paracrine communication with host cells and new matrix formation will mature over time, and therefore the longitudinal assessment in vivo is required to judge the ultimate quality and safety of the desired clinical disease-modifying activity. These therapy aspects cannot be assessed purely with in vitro or computer models, and consequently, animal models are a necessary bridge to human clinical studies and provide the framework for submission, review, and regulatory approval.
A primary obstacle for disc regenerative therapies is whether the product (cells, matrices, and/or growth factors) can stimulate a desirable disease-modifying activity within the inhospitable niche of the degenerating human intervertebral disc (IVD).1 This fact underscores the importance that the chosen animal model mimics the human situation relative to the salient technical and clinical features. The larger the discrepancy between the animal model and human, the greater the risk that the therapy will be ineffectual or even harmful.
Many animal models have been investigated in attempts to clarify disease mechanisms.2,3,4,5 However, these are not necessarily appropriate for judging the quality of a regenerative response. Similarly, there is a diversity of animal models used for preclinical testing of bioactive disc therapies.6 Yet, significant gaps between outcome measures in animals and pain mechanisms in humans muddle decision making when designing preclinical studies. The purpose of this review is to summarize factors that should be weighed when choosing an animal model for therapy development and validation.
5.2 Anatomy
Across the various species used for spine research, the IVD comprises the same basic subtissues: nucleus pulposus (NP), annulus fibrosus (AF), and cartilage end plate (▶ Fig. 5.1, ▶ Fig. 5.2, ▶ Fig. 5.3). This is not surprising given the similar developmental origins and functional requirements to facilitate spine flexibility while supporting load. However, species diversity, principally in size and bipedalism, leads to differences that can become significant in the context of disc tissue engineering.
Fig. 5.1 Safranin-O stained mid-sagittal sections of various animal spinal discs. Scale bars indicate the range of disc sizes. In addition to size, images demonstrate diversity in several features, including nucleus/annulus distinction and presence of a vertebral growth plate.
Fig. 5.2 High power view of Safranin-O–stained disc sections highlighting the interface between the nucleus, cartilage end plate, and subchondral bone. Species differences in end plate cartilage thickness are apparent.
Fig. 5.3 High power view of Safranin-O–stained disc sections highlighting the cellularity of the nucleus pulposus. Species differences in cell type, density, and matrix homogeneity are notable.
5.2.1 Size
There is a tremendous range in disc size across species. For example, disc height can range between 0.25 mm for a mouse and up to 11 mm or more for a human (▶ Fig. 5.1; ▶ Table 5.1). However, when disc height is normalized by width as a measure of shape, the differences between animal and human are less extreme, and range between 12% for mouse and 31% for sheep.7 Yet, these size differences have important biomechanical and biological consequences. Three centuries ago Galileo proposed the square-cube law, which states that as a structure’s size increases, its volume (proportional to length cubed) grows faster than its surface area (proportional to length squared). For example, if an animal’s disc size is doubled, then the surface area is increased by a factor of 4, whereas the volume is increased by a factor of 8. In this illustration, the square-cube law indicates that the larger disc may carry four times as much stress and nutritionally support 8 times as much tissue. Assuming that the same biomechanical and biological principles apply across species, then discs need to be redesigned and/or the animal behavior needs to be modified for tissue homeostasis as the animal becomes larger.
5.2.2 Biochemical Composition
Biomechanical properties of spinal discs can be linked to their biochemical composition and size.8,9 Proteoglycans are a major constituent of the disc nucleus and, due to their fixed negative charge, provide the disc the capacity to osmotically swell to resist compressive mechanical forces.10 Comparative tests indicate that nuclear proteoglycan and water contents are relatively similar between species (▶ Table 5.1), with the exception of rattail, which is significantly less, and baboon disc, which is significantly more.11 Interestingly, despite the range of measured values, proteoglycan and water contents do not correlate with compressive properties across species.
The annulus is reinforced with collagen fibers that are oriented to support disc compression, bending, and axial torsion.12,13 Disc collagen within the nucleus is similar between baboon, goat, and sheep disc, whereas that from pig is less than half and that from calf and cow is more than 3 times greater (▶ Table 5.1).14 Similar variations are noted for the AF, where goat had 48% less and calf had 45% more than human. Yet, like proteoglycan and water composition, collagen content of annulus does not correlate strongly with disc torsional properties.
5.3 Biomechanics
Functionally, disc size, shape, and biochemical constituents affect biomechanical properties, which in turn, can influence how the regenerative effects of biological therapies observed in animals are scaled to judge an effect size for humans. Axial compressive stiffness varies between 13 N/mm in mouse and 2, 491 N/mm in calf spines.11 These differences can be narrowed by normalizing by a ratio of disc height to area, in which case disc stiffnesses from calf, cow, pig, baboon, sheep, rabbit, and rat are not statistically different from human.11 Similar results have been reported for discs tested in axial torsion, where the normalized torsional stiffness (normalized by height divided by the polar moment of inertia) of discs from goat and mouse (tail and lumbar) were within 10% of human, whereas calf and bovine discs were within 25%.14 In this case, however, discs from pig and sheep were approximately 3 times stiffer, perhaps due to differences in annular material properties.15 Taken together, these data indicate that the biomechanical effects of regenerative therapies as measured in animals may be scaled to human as an indicator of anticipated clinical effect size.
5.4 Transport
Because the disc is avascular, disc cells rely on transport to and from capillaries at the outer annulus and vertebral end plate.16 This constraint creates opposing gradients of nutritional factors (e.g., glucose and oxygen) and products of cell metabolism (e.g., lactate17) from the disc periphery to the disc center. The square-cube law makes clear that the surface area for transport becomes disproportionately small compared with tissue volume as the disc size increases. Another consequence of size differences between species is the disc tolerance for metabolic demand. Computational studies demonstrate that the kinetics of solute transport into the disc significantly vary between species in relation to disc size (▶ Fig. 5.4).18 By analogy, diffusion of disc metabolic products out of the disc will have a similar size dependency. These transport dynamics limit the extent of metabolic activity that can be supported within a disc of a particular size. This principle is beautifully depicted by experimental data for nucleus cell density versus disc height across species (▶ Fig. 5.5).19 Importantly, square-cube law scaling effects also explain why smaller discs have a greater healing capacity, as wound-site transport is better able to supply signals and a regenerative milieu, as seen in species-dependent healing of long bone fractures (▶ Fig. 5.6).20
Fig. 5.4 Computational predictions of the time course of glucosamine uptake within the intervertebral disc after intravenous administration. Data indicate that disc size has a large effect on the postinjection concentration profiles. Adapted from Motaghinasab et al.18
Fig. 5.5 Disc cell density as a function of disc size across several species. Red line indicates a curve fit proportional to disc height cubed, supporting that square-cube law scaling influences disc cell homeostasis. Adapted from Stairmand et al.19
Fig. 5.6 Comparison of the time course of long bone fracture healing in mouse, rat, sheep, and human. Adapted from Garcia et al.20
5.5 Surgical Considerations
Variations of disc size across species have additional practical implications. A major disadvantage of small animals (mouse, rat, and to some extent rabbit) is that surgical techniques are limited, and it may not be meaningful or practical to study therapies that involve implants. It may also be difficult in small discs to reliably inject cells that may include viscous carriers (e.g., the mouse nucleus is approximately 0.13 μL11). Injecting through small-gage needles can damage cells due to high shear stress, as lower cell viability is associated with small needle diameter and long needle lengths.21 Also, working with small volumes can lead to proportionately greater errors in the administered dose due to cell adherence within the syringe.
Size constraints are also a factor when attempting to implant tissues, scaffolds, and devices that are part of the regenerative therapy. Scaling to constructs appropriate for small animals may require significant deviation from the formulation and configuration of implants intended to be used in the clinical situation. For this reason, primates have been a model of choice for more traditional spinal implants and, more recently, disc arthroplasty devices.22 Here, disc dimensions are more similar to human and hence more accommodating for implantation. Primates may also be valuable for refining the surgical approach and associated instrumentation.
Another factor is the availability of imaging to facilitate longitudinal studies. The use of magnetic resonance imaging (MRI) to assess disc healing longitudinally can help increase statistical power and reduce cost by using each animal as its own control. However, high field strength is needed to achieve a reasonable resolution in small animals (up to 7 T) and access to imaging resources may be limited for large animals.
5.6 Bipedalism
In addition to differences in metabolic stress between species, there may also be differences in physical loading. Although the common notion is that quadrupeds have lower spinal forces than humans due to their horizontal spine orientation, research in evolutionary biology indicates that in vivo biomechanical stresses are generally independent of animal size, and that tissues across species seem to be operating with a similar factor of safety (ratio of failure load to typical functional load)–of between 3 and 5.23
All animal spines are loaded by ligament and muscle forces developed during movement, and during maintenance of posture against gravitational loading. Even though the spines of quadrupeds are aligned parallel to gravitational forces, significant muscle forces are generated to support bending and torsional movements required for locomotion.24 The observation that vertebral strength24 and normalized disc stiffness (cited above) in animals are comparable to, if not greater than human, also supports the notion that quadruped discs are subjected to significant in vivo force.
Some direct in vivo measurements have been made. Spine forces in baboon are generally proportionate (by body weight) to human, 25 whereas those in sheep are approximately 50%.26 Yet, the intradiscal pressures in sheep can be significantly higher than human depending on the activity.26 Similar to baboon, bovine measurements show that loads and pressures are comparable to human during static postures, whereas dynamic pressures tend to be higher.27
5.7 Cells
Cells are the heart of biological approaches for spinal disc repair. Although the therapeutic strategy may include delivery of donor cells, a bioactive agent that stimulates host cells, or a combination of both, the ultimate goal is to exploit a cellular disease-modifying activity. Several animal model features can impact the behavior of such strategies, potentially in ways that differ significantly from the human situation.
One main consideration is the fact that nucleus cells in animals may be notochordal, and have behaviors that differ from adult human NP cells. During embryonic development of the spinal column, the NP is formed by notochordal cells (NCs) that are sequestered from the developing vertebral bodies and AF.28 Rodents, rabbits, and other nonchondrodystrophoid species3 maintain a high NC number throughout their lifetime, whereas in other animals such as bovine, goat, and sheep, and in humans these cells disappear early in life29,30,31 (▶ Table 5.2).
NCs are histologically and functionally distinct from human NP cells, appearing physaliferous or vacuolated. NCs express phenotypic markers such as CD44s, galectin 3, vimentin, cytokeratins 8 and 19, chondroitin-sulfate proteoglycan (CSPG), and collagen type IIA.29
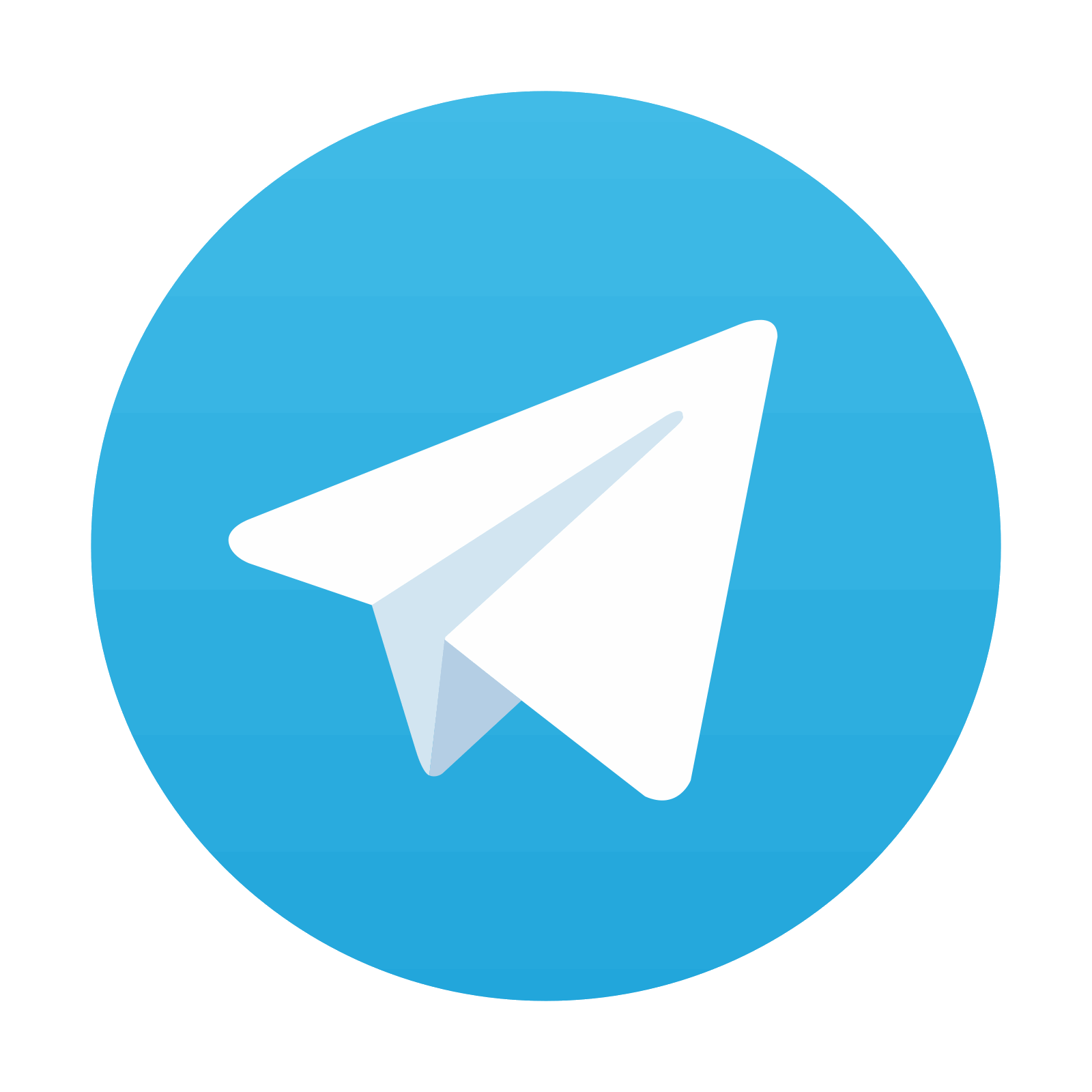
Stay updated, free articles. Join our Telegram channel
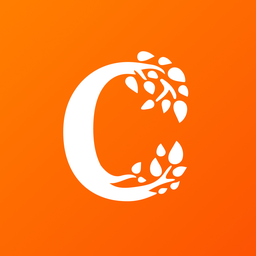
Full access? Get Clinical Tree
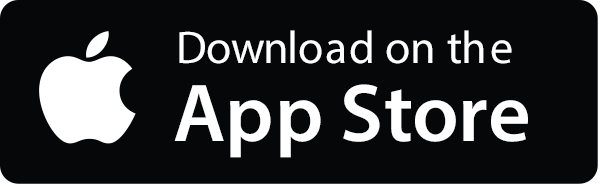
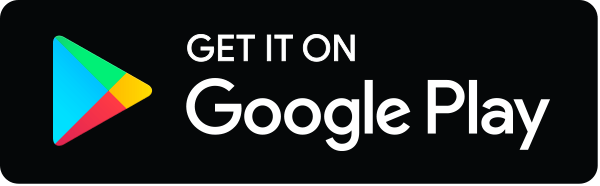