7 Disc Regeneration: In Vitro Approaches and Experimental Results
John T. Martin, Harvey E. Smith, Lachlan J. Smith, and Robert L. Mauck
Abstract
There has been substantial progress toward disc regeneration in the past years and an array of promising strategies have been developed. In vitro culture systems are fundamental to the development and validation of these regenerative therapies, and serve as a prerequisite for preclinical animal research and ultimately clinical translation. This chapter describes the in vitro methods used to evaluate disc regeneration strategies in terms of the cell sources (i.e., pluripotent, multipotent, fully differentiated), the platforms (i.e., monolayer culture, three-dimensional, organ culture), and the external variables for eliciting a cell response (i.e., oxygen tension, pH, glucose, inflammatory factors, mechanical loading, growth factors). Using these in vitro systems, we have learned much about disc cell biology and biomaterials, and have generated a foundation for future research that will inform the implementation of these strategies to rescue disc disease.
Keywords: growth factors, hydrogels, mechanical loading, organ culture, scaffolds, stem cells, tissue engineering
7.1 Historical Perspective
Restoring degenerated or injured intervertebral discs (IVDs) by rescuing or replacing damaged tissue, reestablishing mechanical function, and developing homeostasis in the challenging disc microenvironment is the fundamental goal of IVD regeneration. The last decade has demonstrated marked progress in this field, including the delivery of growth factors and cell-based therapeutics, improvements in bioactive and cell-laden materials for annulus fibrosus (AF) and nucleus pulposus (NP) replacement, and the rise of composite materials for total disc tissue engineering. In vitro evaluation of these strategies is required to address many of the fundamental questions regarding disc repair and regeneration. For example, early in vitro studies focusing on growth factor and cell therapies generated critical data that led to clinical trials, whereas tissue engineering strategies have been advanced from basic work on biomaterials and engineered tissue replacements to implementation in various translational animal models. Finally, new methods of whole disc organ culture and the advancement of bioreactor technologies allow for the evaluation of disc biology and degeneration therapies in the disc microenvironment ex situ. Although these techniques have demonstrated early success, a number of fundamental hurdles still exist before effective, long-term tissue regeneration for early- and late-stage disc disease can be realized. This chapter highlights current in vitro methods for developing and validating regenerative strategies, and outlines persisting challenges in the realization of this important goal.
The first section of this chapter discusses the current clinical state-of-the-art treatments for symptomatic disc disease and provides an overview of experimental regeneration strategies now under investigation. This provides a framework in which to review current in vitro tissue regeneration and replacement methods. Subsequent sections describe the in vitro model systems designed to evaluate regeneration techniques in terms of cell sources, biomaterials, growth factors, and culture methods, and discusses relevant findings generated from each of these techniques. Finally, the outstanding issues and limitations of current regeneration strategies are summarized to provide a foundation and outline for future research in this area.
7.2 Overview of Regeneration Strategies
Although spinal fusion and total disc arthroplasty have seen increasing use in recent years, these interventions do not restore normal spine function; in both cases, the disc is removed and the original structure and mechanical functions of the spine are not replicated. Consequently, while these interventions may initially relieve pain, they are subject to postoperative complications and may not constitute a long-term solution for disc disease. Disc degeneration presents clinically as a spectrum ranging from mild to severe, with the level of tissue degradation correlating to the age of the individual.1 Emerging regenerative strategies are thus calibrated to these stages of disease, with the aim of rescuing the disc from either early- or late-stage degeneration. These methods promise to improve upon current techniques by returning the intervertebral joint to a healthy state. Two general strategies have emerged for treatment at different points along the spectrum of IVD disease.
1.Injectable therapeutics. For early-stage degeneration, where the intrinsic ability of the tissue to repair itself may still be intact but in need of supplementation, the injection of therapeutics into the native disc may slow or even reverse the degenerative cascade. These techniques are aimed at directly improving the quality of the NP, the disc region in which degenerative changes often first manifest. Research on injectable therapeutics is largely based either on the injection of cells to replenish the diseased tissue, or the injection of growth factors that can directly stimulate anabolic behavior of endogenous cells. Additionally, the delivery of anti-inflammatory agents has been proposed to attenuate local inflammation and provide a microenvironment more conducive to regeneration by endogenous or exogenous cells.
2.Total or partial disc replacement. For end-stage degeneration when the native tissue likely has little capacity for regeneration, the removal of the native diseased AF and/or NP tissues and their replacement with an engineered substitute may restore healthy joint structure and function. Efforts to replace the native disc structure through the development of viable engineered tissues leverage the growing knowledge base on cells, biomaterials, and their interactions.
The basic science that underlies each of these approaches is discussed below.
7.2.1 In Vitro Culture Systems
To test if cells, growth factors, and biomaterials can function in a regenerative capacity, numerous in vitro model systems have been developed to mimic specific features of the IVD microenvironment. These in vitro model systems are useful for studying basic physiological processes, injectable therapies, and engineered tissue replacements. In vitro model systems generally include a cell source with regenerative potential, a material substrate on which the cells are cultured, and a set of external variables that can be controlled to replicate the disc microenvironment. The following section introduces these three topics and provides examples from ongoing research in the field to present the current state of the art in this area.
7.2.2 Cell Sources
In the field of regenerative medicine, there are a wide variety of cell sources available for therapeutic use. In general, these can be categorized by their differentiation potential, that is, by their ability to assume phenotypic features similar to cells from different tissue types when provided with the appropriate environmental cues.
Pluripotent Cell Sources
Pluripotent stem cells can differentiate into all cell types and form all tissues derived from the three germ layers. Embryonic stem cells (ESCs), for example, are considered pluripotent and there has been some effort to use these as an injectable therapeutic for disc regeneration.2 ESCs require special handling given their phenotype is difficult to direct and maintain. In addition, clinical use of ESCs requires allogeneic cells which are prone to immune rejection and infection. Likewise, the supply of such cells is currently limited and there are well-known ethical issues related to obtaining fetal cells.
As an alternative to ESCs, fully differentiated cells, such as skin fibroblasts, can be induced into pluripotency by introducing a series of reprogramming genes through viral transduction and other mechanisms.3 These are aptly named induced pluripotent stem cells or iPSCs. Similar to ESCs, iPSCs require a fibroblast feeder layer and a number of chemical factors to first maintain the cells in an undifferentiated state and then to induce differentiation. In contrast to ESCs, however, there is an unlimited supply of donor cells from adult skin tissue. This is a promising, although new, line of research for the regeneration of the IVD4,5 as well as other musculoskeletal tissues.6,7 One drawback of iPSCs is that their preparation is time-consuming, requiring months to generate, differentiate, expand, and direct toward skeletal lineages,8 which may limit their use as an autogenous source. To overcome this, researchers in Japan, Europe, and the United States are developing iPSC banks with fully differentiated cell lines for on-demand access.
Multipotent Cell Sources
There are a number of multipotent stem cell sources under investigation for disc regeneration. Multipotent cells have limited differentiation potential compared with pluripotent cells, but have more clinical relevance given that they can be harvested from adults. For applications in orthopaedics, there are multipotent sources with the ability to adopt phenotypes of a number of musculoskeletal tissues,9,10 including cartilage and fibrocartilage, such as the NP and AF. Mesenchymal stem cells (MSCs), for example, are a popular source because they can be isolated from a number of locations, most commonly bone marrow, adipose tissue, and synovium, and can generate tissues with compositional and functional properties similar to native cartilage.11,12,13 There is a growing body of work on MSCs for disc regeneration that ranges from in vitro experiments to animal models to clinical trials.14 A minor surgical procedure is required in most cases for retrieving MSCs from the donor, and so donor site morbidity remains an issue. Likewise, it is not clear that every MSC has the same potential, and so methods to sort and use optimized subpopulations of MSCs is an area of considerable interest.
Notochordal Cells, Nucleus Pulposus Progenitor Cells
Another source of cells relevant to regeneration are cells that reside within the disc at early development stages that are notochordal in origin. During development, the NP forms from the embryonic notochord,15 and cells that retain a notochordal-like phenotype make up a portion of the NP cellular composition early in life. The disappearance of these cells, potentially due to either differentiation into less metabolically active chondrocyte-like cells or apoptosis, may be associated with the onset of degenerative change at older ages. Thus, it has been hypothesized that notochordal cells play an important role in disc homeostasis and may have regenerative properties. Currently, there is significant research interest in notochordal cells for disc regeneration,16,17,18,19,20,21,22,23,24,25 either through direct injection into a degenerate disc, or for use in co-culture with another cell source such as MSCs or degenerate NP cells. Although studying the function of notochordal cells will likely elucidate mechanisms for regenerative therapies, a reliable source of notochordal cells, one that can be harvested and expanded for injection or tissue engineering, has yet to be identified.
Fully Differentiated Cell Sources
Fully differentiated cells also have the potential for regeneration, despite their terminally differentiated state. It has been well demonstrated that AF and NP cells can be isolated and cultured in vitro, and that these cells can produce tissue whose composition largely mirrors that of the native extracellular matrix (ECM); gene expression data suggest that AF cells maintain a fibrochondrogenic phenotype, with high levels of types I and II collagen messenger RNA (mRNA); the NP phenotype is more chondrogenic and, similar to articular chondrocytes, NP cells express mRNA-related type II collagen, the chondrogenic transcription factor SOX9, and aggrecan.26,27 NP cells also express unique factors that likely reflect their notochordal origin and the unique microenvironmental niche in which they must survive and function.28 Potential sources for therapeutic AF and NP cells are from discarded disc tissue, such as degenerate tissue removed prior to spinal fusion for spondylolisthesis/disc disease. However, there exist technical hurdles related to this cell source given that degenerate tissue yields cells with an altered phenotype characterized by decreased proteoglycan production, senescence, catabolism, and a number of inflammatory markers.29,30,31,32 However, these cells may be rescued from their degenerate state prior to their injection into the disc space, for example, by co-culture with a healthy cell population.33,34
Other terminally differentiated cells can be sourced from cartilage at various anatomical locations; these cells have demonstrated potential for musculoskeletal regeneration as well. Articular chondrocytes from non–load-bearing regions of the knee have been widely used for cartilage restoration procedures such as autologous chondrocyte implantation.35,36 Whereas these cells have robust chondrogenic potential, their isolation is associated with local tissue damage. Nasal37 and auricular38 chondrocytes have demonstrated potential for in vitro chondrogenesis, and local donor site morbidity related to the isolation of these cells may be preferable when compared with articular chondrocytes because they are not in an environment that has potential for joint-level communication of inflammatory signals, as is the case for a synovial joint. Additionally, allogeneic articular chondrocytes have recently become commercially available. As these cells are prepared from juvenile (deceased) donors, this is a source of highly active chondrocytes that has shown some clinical success in treating articular cartilage defects39; these cells may likewise have potential for disc regeneration applications.
7.2.3 Culture Systems
The physical environment in which cells are cultured has a significant influence on experimental outcomes; cells read cues from material substrates to regulate phenotype and metabolic activity.40,41 The standard material platforms for disc cell culture fit into one of three categories: cells cultured in a thin layer on plastic dishes (monolayer culture); cells aggregated as pellets, encapsulated in hydrogels, or seeded onto fibrous scaffolds (three-dimensional [3D] culture); live disc explants removed from animals or human cadavers and cultured in the lab (organ culture) (▶ Fig. 7.1).
Fig. 7.1 In vitro model systems. (a) Monolayer culture allows for simple high-throughput studies of cell behavior. (b) Organ culture allows for studies of disc cells in their native environment. AF, annulus fibrosus; VB, vertebral body; VEP, vertebral end plate. (c) By removing the bulk of the vertebral body, the disc can remain viable in culture. This allows for the study of disc cells in their native environment. (d) To simulate the AF, cells can be cultured on scaffolds that mimic the organization of collagen fibers found in the AF region. (e) To simulate the nucleus pulposus (NP), cells can be cultured in hydrogels so as to reproduce a spherical cell morphology. (f) A combination of fibrous AF scaffolds and hydrogel NPs allows for co-culture of cells in a simulated disc environment.
Monolayer Culture
Due to ease of manipulation and experimental assays, simple monolayer culture conditions have been used to generate the majority of our knowledge regarding mammalian cell behavior, and IVD cells are no exception. The procedure for culturing disc cells or other cell types in monolayer involves first isolating cells from donor tissue, suspending these cells in a growth medium, and plating the cell suspension onto a sterile polystyrene culture dish. Cells attach directly to the dish and can be serially passaged to expand their number. A number of outcomes can be measured; cells can be retrieved and their RNA can be extracted for gene expression analysis; cultures can be directly stained for protein and ECM components; cell appearance and morphology can be evaluated by microscopy; culture media can be extracted and its composition can be analyzed.
One or more cell types and their interactions can be evaluated by simple modifications to a monolayer culture system. For example, the influence of NP cells on AF cells can be evaluated through co-culture; NP and AF cells can be seeded in direct apposition to study the influence of direct cell–cell communication, or seeded in culture dishes that have two tiers (one of which is porous) to study the influence of paracrine signaling between these two cell types. These are popular techniques in disc regeneration to evaluate the influence of one cell type on another, for example, to generate an NP-like phenotype in MSCs, MSCs can be co-cultured with NP cells.42
Three-Dimensional Culture
In vivo, disc cells reside in a three-dimensional microenvironment that is not well represented by monolayer culture systems. Phenotypic differences are evident when comparing NP cells cultured in monolayer, where they develop a fibroblast-like phenotype, with NP cells cultured in a 3D environment.43,44 Indeed, the “de-differentiation” that invariably occurs during monolayer cell expansion complicates interpretation of data acquired using monolayer culture methods.
A more realistic culture system would provide 3D spatial cues for cells to promote or preserve their phenotype, and although the types of experimental assays suitable for application to cells in 3D culture are more limited than for monolayer culture, advantages include the ability to more effectively assess matrix elaboration and mechanical function. In vitro NP and AF models can be scaffold-free (as in pellet culture) or can be generated from naturally occurring (agarose, alginate, collagen, fibrin, and hyaluronic acid [HA]) or synthetic (polyethylene glycol, polyvinyl alcohol, polylactic acid, and polycaprolactone) biomaterials (▶ Fig. 7.2a). Material topographies include homogeneous materials, composite networks of a bulk polymer and an additional interpenetrating polymer, and aligned and randomly oriented fibrous scaffolds. These materials are typically cross-linked to infer stability to the polymer network; this can be initiated through photo, thermal, and chemical stimuli, and depending on processing parameters, can lead to sparsely or densely cross-linked networks to enable tight control over the physical properties of the bulk material. Additionally, direct regulation of cellular phenotype and adhesion can be exerted by including growth factors and adhesion ligands that mimic natural ECM proteins (▶ Fig. 7.2b). As cell phenotype is driven by mechanical cues, biomaterial substrates selected for AF and NP cell studies often reflect the physical properties of the native NP and AF; soft hydrogels that induce an NP-like phenotype are used for NP cell studies, whereas fibrous scaffolds that instruct seeded AF cells to elongate and develop a fibrochondogenic phenotype are often used in AF cell studies.
Fig. 7.2 Three-dimensional culture systems: material selection, fabrication, and examples. (a) A variety of naturally occurring and synthetic biomaterials, with specific physical and chemical properties, are available individually or as composite materials as a framework for cell culture. (b) Fabrication processes allow control over structural features and physical properties, and can incorporate bioactive components to better match the intervertebral niche or to elicit a desired cellular response. (c) In vitro nucleus pulposus (NP) model: NP cells cast in hyaluronic acid hydrogels develop a disc-like phenotype over 8 weeks of culture. This is evidenced by increasing levels of type II collagen and glycosaminoglycans (GAGs; scale = 100 μm).54 (d) In vitro annulus fibrosus (AF) model: (Top left) scanning electron microscopy (SEM) images of electrospun scaffold with aligned and random orientations (scale = 10 μm). (Bottom left) Mesenchymal stem cells seeded on aligned scaffold demonstrate preferential alignment in the fiber direction (scale = 20 μm). (Top right) En face transmission electron microscopy (TEM) image of cell bodies aligned with the fiber direction (a cell nucleus is outlined with dotted white line; scale = 10 μm).111 (Bottom right) Cross-sectional TEM image of a cell anchored at two electrospun fibers (starred). Collagen fibrils deposited by the cell (dark puncta) populate the space between the cell and the electrospun fibers (scale = 10 μm).111,112
Pellet Culture
Cells in a high density suspension can be centrifuged and concentrated in a pellet, forming a spheroid scaffold-free cell aggregate. Cell-generated ECM accumulates in the pellet over time, forming a physiological 3D microenvironment. Pellet culture is often used as a simple and more realistic alternative to monolayer culture to study basic cell functions, such as their response to inflammatory factors and hypoxia.45 Additionally, scaffold-free aggregates are emerging for tissue engineering applications,46 where multiple pellets can be combined into large structures, or aggregation geometry can be controlled to match a defect shape such as those common to the articulating surface of the tibia. One could envision exploiting this method for disc tissue engineering, though these studies have not yet been conducted. One drawback of the pellet culture approach is that, in most connective tissues, cell density is low and cell–cell contact is not common; thus, high density cell aggregates produce abnormal cell–cell contact that may influence experimental findings.
Hydrogels
For 3D culture systems specific to the IVD, hydrogels are often preferred for NP cell studies (▶ Fig. 7.2c); the physical cues provided by soft hydrogels tend to induce a spherical cell shape and promote an NP-like phenotype. In contrast to pellet monolayer culture, hydrogel encapsulation provides a 3D environment that better mimics the native NP environment and limits cell–cell contact.
There are a number of applications in NP regeneration in which hydrogels can be useful. Hydrogel culture systems allow for studying basic cell responses; for example, hydrogel systems are used to determine how NP cells respond to inflammatory challenge and anti-inflammatory interventions.47 In a very active area of study, hydrogel vehicles are used to deliver cells for NP regeneration.48,49,50,51,52 Hydrogel-mediated cell delivery serves as an alternative to delivery in a liquid carrier (such as saline or media), and physical parameters such as viscosity of the hydrogel can be tuned to both protect the cells during delivery as well as improve their retention at the delivery site. In addition, the chemistry of the hydrogel may be exploited to modulate cell activity; for example, a polyethylene glycol hydrogel can be modified to include cell adhesion ligands designed to influence cell phenotype.53 Alternatively, the backbone of these hydrogels may be designed based on naturally occurring materials within the disc; another study demonstrated that the encapsulation of NP cells in HA, a ubiquitous ECM component, drives cells to express NP-specific markers.54 Due to their inherent physical properties, acellular hydrogels may allow for functional restoration by restoring native tissue mechanical properties in the disc space.55,56 This restitution of disc mechanics may have a regenerative impact on endogenous cells by normalizing stresses and strains that they experience.
Whereas hydrogels are largely used for the study of NP cells, they have also been used for AF regeneration studies. To study basic cell functions, AF cells can be encapsulated in hydrogels; for example, AF cells in agarose respond to growth factor stimulation and osmotic loading.57 Others have also taken advantage of cell-mediated remodeling of hydrogels to build engineered AF-like tissues; fiber alignment can be generated by depositing cell-laden collagen around a post and allowing the gel to contract, producing circumferential fiber architecture similar to the native AF58; adhesive gels are also in evaluation for the repair of AF fenestrations that remain after microdiscectomy.59,60
Fibrous Scaffolds
Given the ordered structure of the AF, scaffolds composed of aligned polymer fibers, of geometry ranging from nanoscale to microscale, are preferred for AF tissue engineering (▶ Fig. 7.2d). These scaffolds provide a topographical template; when cells are seeded on fibrous scaffolds, they will orient and elongate in the prevailing fiber direction. These topographical cues direct cells toward a phenotype similar to that of AF cells,61,62,63 depositing ordered ECM that acts as a mechanical reinforcement in the fiber direction. The hierarchical fiber structure in the AF, with alternating angles ± 30 degrees in apposed layers, can be constructed from sheets of aligned fibers using a layering technique,64 and this structure can be maintained after in vivo implantation.65 This methodology has also been exploited to develop engineered fibrous tissues composed of MSCs with mechanical properties matching that of the native AF.66
Composite Disc Constructs
Beyond simple realization of the component parts of the disc, recent research efforts have expanded to include the ambitious goal of total disc replacement with an engineered, cellularized artificial disc, combining hydrogel NP regions with fibrous AF regions (▶ Fig. 7.3). This methodology allows for the evaluation of NP and AF cells in co-culture, and for the evaluation of other potential cell types like MSCs. It also allows for the interrogation of total disc mechanical properties, which are of particular importance for engineered total disc replacement. NP and AF regions that comprise the total disc constructs have taken a number of forms with varying levels of complexity, ranging from 3D-printed polymer discs,67 to nanofibrous scaffold AFs wrapped around hydrogel NPs,64 to collagen gels with circumferential fiber alignment about a central hydrogel NP region.68 Even more recently, this work has been extended to include engineered end plates into total disc constructs to improve potential attachment at the vertebral junction.69
Fig. 7.3 Composite engineered discs. Engineered discs are fabricated from a variety of materials and cell types. (a) Silk annulus fibrosus (AF), fibrin and hyaluronic acid nucleus pulposus (NP), porcine disc cells and chondrocytes113; (b) poly (glycolic acid) AF, alginate NP, ovine disc cells114; (c) poly(e-caprolactone) foam AF, hyaluronic acid NP, bovine disc cells or mesenchymal stem cells (MSCs) (unpublished work from our lab); (d) electrospun poly(e-caprolactone) AF, alginate NP, bovine disc cells or MSCs64; (e) collagen AF, alginate NP, ovine disc cells58; (f) demineralized bone matrix gelatin AF, collagen, hyaluronic acid, chondroitin sulfate NP, lapine disc cells115; (g) poly(glycolic acid) AF, alginate NP, ovine disc cells.116
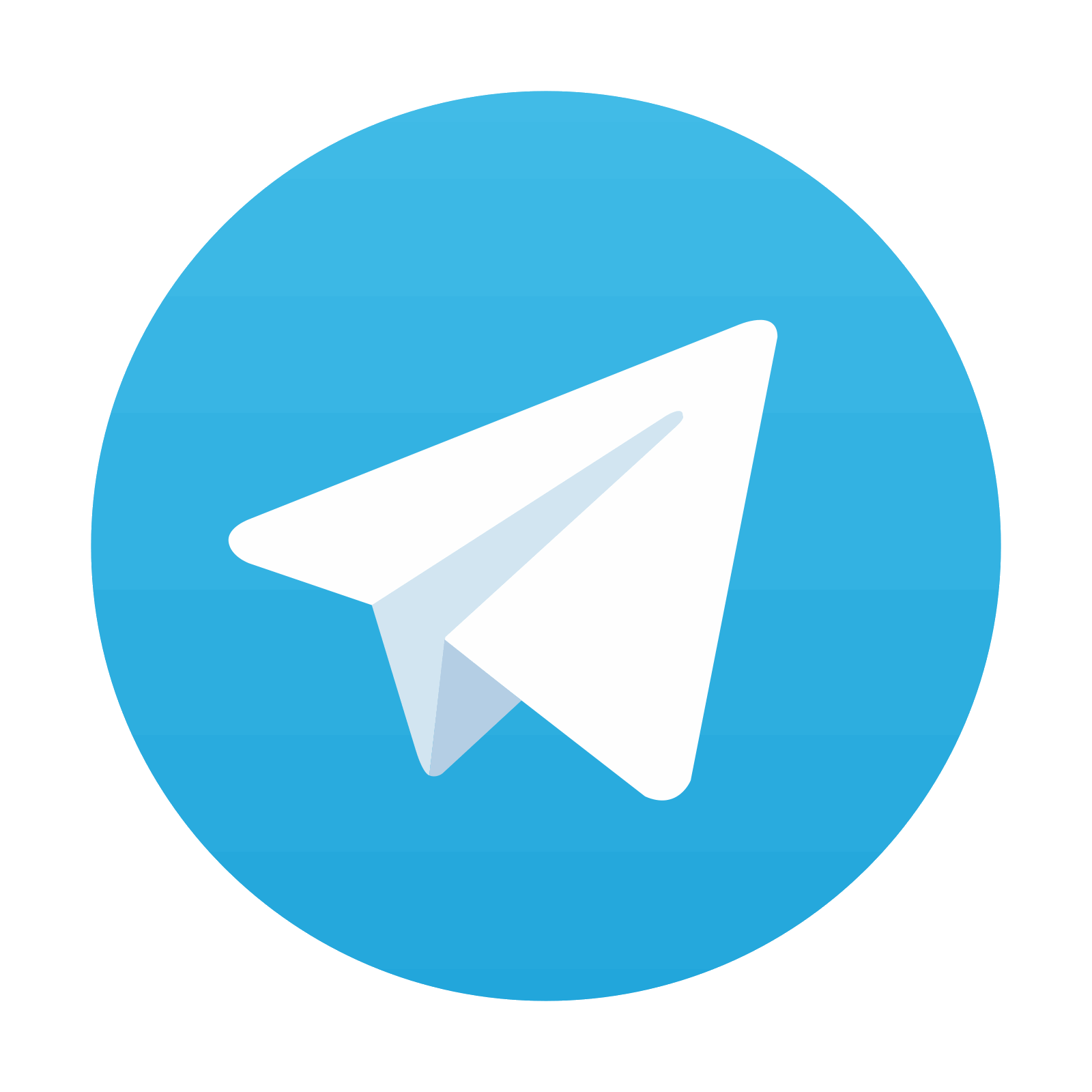
Stay updated, free articles. Join our Telegram channel
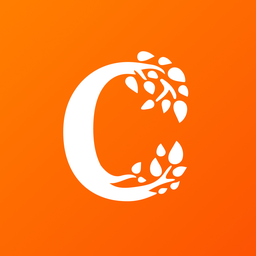
Full access? Get Clinical Tree
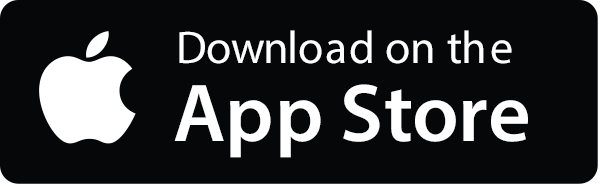
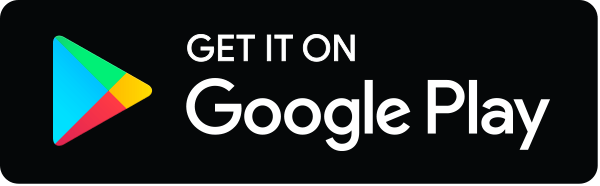