, George F. Koob1 and Leandro F. Vendruscolo1
(1)
Committee on the Neurobiology of Addictive Disorders, The Scripps Research Institute, 10550 North Torrey Pines Road, SP30-2400, La Jolla, CA 92037, USA
Abstract
Animal models of pain play an important role in the characterization and development of therapeutics for several pain conditions. Models that mimic the development and maintenance of chronic pain conditions are especially important and have directly impacted the availability of treatments for many common pain conditions such as neuropathic, inflammatory, and visceral. The most efficacious and first choice treatment option for chronic pain is opioid analgesics. Besides being potent analgesics, opioids produce an intense pleasant feeling (reward), especially in individuals not suffering actual pain. This raises the concern that the chronic treatment of pain with opioids can cause opioid tolerance and dependence. This chapter discusses the animal models that are used to quantitatively measure nociception and analgesia, and animal models that are used to study inflammatory, neuropathic, and visceral pain. Additionally, we discuss the animal models used to study opioid dependence.
Introduction
The most pervasive and universal form of human distress is pain. It has an inverse relationship with one’s quality of life [1, 2] and negatively impacts collective social productivity [3]. Pain is one of the most common reasons for a visit to a physician’s office, community health center, and emergency rooms and is now considered the fifth vital sign. When pain interferes with and at times halts the activities of one’s daily activities, pain becomes central to that person’s daily existence.
While most acute pain can be easily treated and is essential for disease diagnosis, conversion to a chronic pain condition often becomes more difficult to treat and retains little value in terms of identification of pending damage, dysfunction, or disease. Such chronic pain conditions become challenging to treat for a variety of reasons. First, chronic pain can arise from a wide variety of central or peripheral nervous system dysfunctions such as inflammation, neuropathy, disease specific, or related to cancer. In many cases, the pain is caused by multiple points of dysfunction. Such conditions are often difficult to treat pharmacologically [4–6] for reasons associated both with the neurobiology of pain and the chemistry of pharmacological agents used to treat pain. Specifically, chronic pain of differing etiology may evoke differential neurochemical and molecular changes at multiple levels of the central nervous system [7]. These alterations often occur at the neurotransmitter (e.g., endorphins, substance P, glutamate, etc.) and/or receptor (e.g., opioid, neurokinin, glutamate receptors, etc.) levels. The complexity of pain processing systems presents a challenge for pharmacological interventions for pain relief. For this reason, there currently are fairly limited options for pharmacological treatments for chronic pain. That is an area of universal disappointment for pain researchers, industry, practitioners, patients, and their families.
The primary and most effective class of pharmacological agents used to manage chronic pain remains the opioid agonists. Opioid receptors are inhibitory G protein-coupled receptors expressed at nearly every point in the pain signal conduction pathway (primary afferent peripheral nerve terminal, primary afferent spinal terminal, second order neurons, rostral ventral medulla, periaqueductal gray, and thalamus). Therefore, they are well positioned to regulate the pain signal and appear to be the most effective target for this purpose. Consequently, opioids have been used for centuries to control pain. However, opioid receptors are also expressed in brain regions that control emotion and reward, which can be dysregulated with chronic drug exposure and lead to drug dependence and addictive behavior.
For many years, the claim was made that chronic pain patients, who take opioid medication for treatment of their pain, self-administer the drug to attain the analgesic effect and rarely become addicted [8]. It was on that basis that prescribers have been increasingly educated and retrained to appropriately treat patients for their pain, which is often undertreated; it has been asserted that only 25 % percent of chronic pain patients receive adequate treatment [9]. Concerns were raised regarding practitioner bias against prescribing opioids starting at the level of medical school students [2] and other health professionals such as pharmacists and regulators [10]. It is noteworthy that among medical board regulators, the perceived legal and medical acceptability of treating patients with a history of opioid misuse, even for cancer-related pain, was significantly reduced relative to patients without a history of misuse [10]. The introduction of sustained-release opioids to the pain management armamentarium in the 1990s provided extensive initial enthusiasm [11] in terms of improved pharmacokinetics in that these new sustained-release formulations offered serum levels of opioids that could be maintained at steady state for 12–24 h. Such a pharmacokinetic profile meant that patients needing chronic pain management would be at significantly less risk for breakthrough pain associated with opioid regimens reflecting shorter half-lives that result in more frequent drops in serum levels below the minimal effective concentrations [11]. Therefore, the introduction of sustained-release opioid medications was considered a significant advance in treatment. However, concern regarding opioid therapy for pain management is still controversial for at least two reasons: the continued perceived misuse of opioids and the lack of efficacy in nonmalignant pain management.
It remains unclear whether there is a biological basis for restricting the use of opioids in patients with chronic pain, particularly of nonmalignant origin. The National Institutes of Health (NIH) has recently acknowledged this problem and has attempted to address this issue by developing research programs specifically to investigate the relationship of prescription opioid use and misuse, CNS changes that occur with chronic pain, and how these changes parallel those that occur with drug addiction. In 2005 and 2008, requests for applications were announced by NIH that specifically addressed these issues with an emphasis on clinical research in the first case Prescription of Opioid Use and Abuse in the Treatment of Pain (NIDA/NIA/NIDCR) and an emphasis on basic research in the second case Central Nervous System Intersections of Drug Addiction, Chronic Pain and Analgesia (NIDA/NINDS).
Research investigating the effectiveness of morphine in neuropathic pain patients showed that morphine was not effective in certain forms of neuropathic pain [12]. However, this study was limited in scope in terms of patient population and dosing schedule. The patients received two doses of 10–20 mg morphine i.v. and were only evaluated 15 min following infusion. In addition, these patients suffered from severe neuropathic pain that had been unresponsive to nerve block, surgery, transcutaneous electrical nerve stimulation (TENS), and drug therapy including other opioids such as buprenorphine and pentazocine. The overall conclusions of the 1988 study are still used to support the argument against use of opioids for neuropathic pain; yet, these results have been challenged by many preclinical and clinical trials [13–15].
Using an animal model of neuropathic pain, La Buda and colleagues examined the efficacy of several pharmacological agents that are used for treatment of neuropathic pain. They found that the nonopioid drug gabapentin, which interacts with voltage-gated calcium channels and increases synaptic availability of γ-aminobutyric acid (GABA), reversed spinal nerve ligation (SNL)-induced neuropathic pain with complete efficacy [14].
Several reports have contributed to the growing acceptance of the assertion that patients with noncancer pain may achieve good pain control from opioid therapy [16]. However, there is also consensus that the current clinical literature on opioid use for the treatment of noncancer pain does not address issues of long-term opioid maintenance and that such studies are critical to inform management plans for treatment of noncancer chronic pain patients with opioid therapy [17, 18].
To provide an evidence-based animal model framework to move the field forward, this chapter discusses the animal models that are used to quantitatively measure nociception and analgesia, and animal models that are used to study inflammatory, neuropathic, and visceral pain. We also discuss the animal models used to study opioid dependence. Finally, we discuss how the use of models of chronic pain and opioid dependence can increase our knowledge on the neurobiology of the association of chronic pain and drug addiction.
Quantitative Measurements of Hyperalgesia
Many models have been developed to mimic human conditions and to study pain and its underlying mechanisms in whole animal systems. These models allow for quantitative and qualitative measurements and examination of alterations of the physiology during the chronic pain state. Animal models of chronic pain are used to (1) induce a pain state that mimics common human conditions of pain, (2) quantitatively characterize the degree of pain resulting from the injury, (3) evaluate mechanisms responsible for pain, and (4) evaluate analgesic efficacy directed toward alleviation of the injury.
Animal models of pain include models of hyperalgesia, defined as increased sensitivity toward painful stimuli and models of allodynia in which normally nonnoxious stimuli are perceived as painful. These models are used to study neuroadaptive changes associated with chronic pain. These measurements, which are the dependent variables, are generally quantified as a deviation from the normal baseline threshold in each animal before induction of injury and the resulting pain. Following administration of potential analgesics measurements assessing hyperalgesia or allodynia can be taken again to evaluate the efficacy and potency of the analgesic of interest.
Hyperalgesia or allodynia can be examined using a variety of models that measure force applied to the affected area or time to withdraw the paw when exposed to a stimulus. It is important to consider the model of pain when choosing the method of hyperalgesia assessment. While hyperalgesia resulting from inflammation can effectively be assessed using mechanical, thermal, and static models, there has been mixed reviews regarding assessment of neuropathic pain. Most often neuropathic pain is assessed using mechanical stimuli and there has been limited data supporting the use of thermal hyperalgesia to assess neuropathic pain. An overview of common measurements is described below.
Mechanical (Von Frey) Paw Withdrawal
Maximilian Ruppert Franz von Frey developed the quantitative measurement of mechanical hyperalgesia. Individual filaments of specific calibrated forces, which determine nociceptive thresholds of individual subjects, were originally designed for diagnostic purposes and later incorporated to animal studies. The use of these filaments for the purposes of quantifying hyperalgesia in rats was described by Chaplan and colleagues [19]. Filaments of varying forces are applied to the plantar surface of the affected paw for a period of 3 s starting with the lowest force and increasing the filament force until the animal withdraws its paw. Following the first withdrawal, the next lowest force is applied. If the animal withdraws its paw the next lowest force is applied; if the animal does not withdraw its paw the next highest force is applied and this pattern continues for five applications following the first withdrawal. This pattern results in a threshold that determines the animal’s individual withdrawal threshold. Low withdrawal thresholds indicate a state of hyperalgesia while higher withdrawal thresholds indicate normal (or baseline) withdrawal thresholds. When testing an analgesic for efficacy, a return to high withdrawal thresholds indicates analgesia. This test is typically responsive to opioid analgesics. Figure 5.1 shows that chronic infusion of the chemotherapeutic agent vincristine (see animal models of neuropathic pain) produces mechanical allodynia indicated by low paw withdrawal thresholds. Morphine injected prior to testing substantially increased pain thresholds indicating potent analgesia. The analgesic effect of morphine was reversed by pretreatment with the opioid antagonist naloxone, indicating the participation of μ-opioid receptors on morphine-induced analgesia.
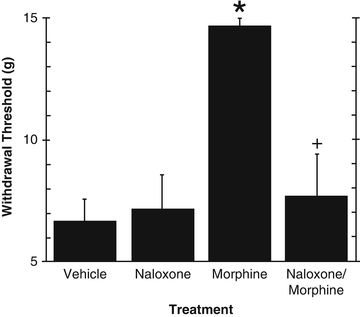
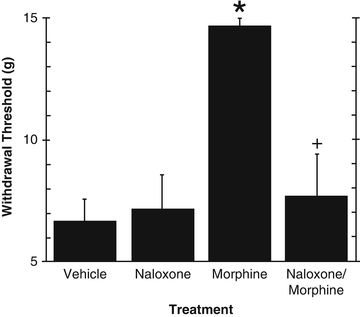
Fig. 5.1
Effect of naloxone hydrochloride or vehicle, administered 15 min prior to injection of morphine sulfate or vehicle, on mechanical allodynia. *p < 0.05 vs. vehicle. + p < 0.05 naloxone/morphine vs. morphine. Taken from Lynch et al. (2004), with permission
Randall–Selitto
Nociceptive thresholds here are determined using a paw-pressure vocalization test by which increasing pressure is applied to the top of the hindpaw until vocalization [20] using an analgesimeter. In general, a 600-g cut-off value is used to prevent tissue damage.
Thermal Paw Withdrawal
Paw withdrawal from a heat stimulus is also used to test hyperalgesia of affected areas. A thermal (light) stimulus is applied to the plantar surface of the affected paw and the time to paw withdrawal is recorded after which the stimulus is removed. The most commonly used apparatus for this test was developed by Hargreaves and colleagues [21]. A shorter latency to paw withdrawal indicates hyperalgesia and an increased time to paw withdrawal indicates either analgesia or normal baseline thresholds and can be calculated with the following formula: [(uninjured paw latency—injected paw latency)/uninjected paw latency] × 100. A cutoff time of 30 s is commonly used to prevent tissue damage from repeated tests.
Weight Bearing
The weight bearing model is not a model of evoked hyperalgesia, but is a static model that measures an important characteristic of most pain states, that of spontaneous or tonic pain. With a unilateral injury, animals will favor the injured area by putting most of their weight on the noninjured paw. Medhurst and colleagues (2000) first described this model [22]. The animal sits in the chamber with each hind paw on a balance that shows the amount of weight an animal is willing to put on each paw. The difference between the two paws (uninjured paw—injured paw) is calculated with the higher difference score indicating hyperalgesia.
Animal Models of Chronic Pain
There are several animal models (the independent variables) utilized to produce a state of hyperalgesia that mimic common ailments such as inflammation (arthritis, burns, and Crohn’s disease, and other causes of acute or chronic inflammation) or neuropathic pain resulting from physical trauma, diabetes, chemotherapy, or from other etiologies. The use of these models has led to many insights regarding the physiology of specific pain states, receptor regulation, and possible treatments.
Inflammation
Animal models of inflammation have taught us important aspects of inflammatory pain that are specific to inflammation and different than pain arising from other origins such as nerve injury. Valuable information regarding recruitment of inflammatory mediators that result in chronic pain and upregulation of sensory transduction mechanisms that lead to sensitization in the periphery and central nervous system have also led to a deeper understanding of how to treat pain from an inflammatory origin. Several receptors respond to inflammation and contribute to hyperalgesia and sensitization. Below we describe the most common animal models of inflammation.
Carrageenan
Carrageenan-induced inflammation can be used as a model of acute inflammation with hyperalgesia occurring 3 h after injection and lasting out to approximately four days [21]. λ-carrageenan is a sulfated polysaccharide that induces an inflammatory response by activating microglial immune responses and activates mechano-heat sensitive C-fiber receptors [23]. Carrageenan is dissolved to a 2 % solution with physiological saline and injected subcutaneously in the plantar surface of the hindpaw in a volume of either 150 μl (rat) or 30 μl (mouse).
Complete Freund’s Adjuvant (CFA)
CFA is a heat-killed mycobacterium that when injected into the hindpaw induces an inflammatory immune response. The advantage to this model is that it induces chronic inflammation that is apparent at 48 h and lasts out to approximately 3–4 weeks [24]. It is most commonly prepared by emulsifying suspended CFA in mineral oil with physiological saline in a 1:1 ratio. The resulting emulsification is injected subcutaneously into the plantar surface of the hindpaw at a volume comparable with carrageenan, 150 μl (rat) or 30 μl (mouse).
Polyarthritis
The polyarthritis model was first applied as a pain model by Colpaert and colleagues in 1980 [25]. CFA is injected into the base of the rat’s tail. Under light anesthesia, animals are injected intradermally with 5 mg/ml of emulsified CFA in a volume of 50 μl. Following an incubation time of 2 days, animals develop widespread joint inflammation. Analysis has shown that chronic pain has been shown to peak between days 18–21 and terminate by days 35–40 [26]. Behavioral effects are similar, in scope, to other models of pain and have been validated by the use of analgesics. Measurements of hyperalgesia include, mechanical thresholds, and thermal paw or tail withdrawal.
Neuropathic Pain
Neuropathic pain is pain that originates from the nerves due to actual or perceived damage (International Association for the Study of Pain). Neuropathic pain can commonly arise from physical trauma, disease states such as diabetes, and as a result of treatment for other diseases, most commonly in conjunction with cancer chemotherapeutics. Tissue damage contributing to neuropathic pain can have lasting effects, following apparent recovery, to the surrounding nerves, which can severely impact quality of life and mobility. In general, neuropathic pain results in allodynia defined as where nonpainful stimuli or activity such as brush or movement become painful. During a neuropathic pain state, there is upregulation of several proinflammatory cytokines and increases in glutamatergic transmission that lead to sensitization of pain pathways.
Because of the unique nature of neuropathic pain, reliable and quantifiable models of neuropathic pain were developed. Each of the described models has unique profiles of nociception. SNL and partial nerve injury result in robust mechanical allodynia whereas chronic constriction injury (CCI) is more sensitive in thermal and cold nociception assays [27]. One specific advantage of these models is that the allodynia is contained to the side of the injured nerve, leaving the contralateral paw unaffected. This allows for a within subject control when evaluating possible analgesics.
Chronic Constriction Injury
CCI involves four loose ligatures of cat’s gut suture around the common sciatic nerve. The suture irritates the nerve and it then becomes inflamed which induces neuropathic pain. This model, first developed by Bennett and colleagues, produces marked allodynia, as evidenced by radiant heat and mechanical withdrawal by day 2–3 and lasts out to 2–3 months [28].
Spinal Nerve Ligation
SNL is frequently used to induce neuropathic pain in rat and mouse and was first described by Kim and colleagues [29]. Tightly ligating around the lumbar nerves, five and six (L5/L6) of the spinal cord produces a robust and reproducible mechanical allodynia in the ipsilateral hindpaw that lasts indefinitely. A ligation around L5 before the nerve converges with the L4 and L6 nerve to become the sciatic nerve spares the anesthesia and paralytic effects associated with tight ligation of the entire sciatic nerve. The adaptation to mouse in which L5 (but not L6) is ligated was first described by Mogil and colleagues [30] in a comparative study on the impact of inbred strain differences on the development of nerve injury-induced hyperalgesia. Similarly, we and others have since observed that ligation of L5 is sufficient to establish tactile hypersensitivity of the hindpaw in rat (personal observations and communications).
Spared Nerve Injury
The spared nerve injury (SNI) was developed by Decosterd and Woolf [31] to elucidate contributions of each part of the sciatic nerve of which the sensory component is comprised of the tibial, common fibular, and sural nerves. SNI is a partial denervation model that provides minimal variability with regard to physical damage to the nerve. In this model, the tibial and common fibular nerves are axotomized leaving the sural nerve intact. Nerves are tightly ligated with 5-0 silk suture and sectioned distal to the ligation removing 2 mm of the distal nerve. This injury results in both mechanical and thermal sensitivity.
Chemotherapy-Induced Neuropathic Pain
Treatment with vinca-alkaloid chemotherapeutics often result in neuropathic pain, which is difficult to safely and effectively treat. Inhibition of microtubule growth leads to disruption of mitosis, which is the intended effect of the halting the progression of cancer; however, this also leads to painful neuropathy due to the effects on microtubules in peripheral nerves [32]. Peripheral neuropathy is often first diagnosed in the hands and feet. The chemotherapy-induced peripheral neuropathy model was first described by Aley and colleagues using vincristine [33] and adapted by Nozaki-Taguchi to eliminate the need for daily injections [34]. The drugs paclitaxel, oxaliplatin, and other vinca-alkaloids [35, 36] have also been used in this model. The intensity of neuropathic pain is dependent on the dose and duration of treatment.
Animal Models of Opioid Reward, Addiction, and Withdrawal
Opioid abuse and dependence are major public health problems. According to the Substance Abuse and Mental Health Services Administration (2010), the number of people dependent on or abusing pain relievers increased between 2002 (1.5 million) and 2009 (1.9 million). Therefore, a major need exists for research into the neurobiology of opioid addiction with the hope of developing better strategies for the treatment of opioid dependence. Treatment options to date are largely limited to substitution-therapy with the use of long-lasting opioid drugs, such as methadone and buprenorphine, or limited use of α2-adrenergic agonists, such as clonidine [37, 38], which all have pronounced adverse effects.
Although addiction (or drug dependence as defined by the Diagnostic and Statistical Manual of the American Psychiatric Association) [39] is a typical human condition, physiological, psychological, and behavioral phenotypes have been described in several animal species and are thought to be analogous to human addiction. Depending on the scientific question to be investigated different models can be used to assess different aspects of drug dependence (e.g., intoxication, withdrawal, relapse, physical dependence, etc.). Here, we describe some of the most commonly animal models used in the addiction field with emphasis on intravenous (IV) self-administration.
Intravenous Self-administration
IV drug self-administration is the most widely accepted model for the study of the role of opioid reinforcement in drug addiction. Drug administration through the IV route results in nearly immediate drug effects and is among the most highly reinforcing delivery routes due to the rapid onset of drug effects. In this model, virtually all drugs with high abuse potential in humans, including prescription opioids, are voluntarily self-administered by laboratory animals. The animals (e.g., rat, mouse, and monkey) are prepared with a cannula into a vein (typically the external jugular vein) for direct access of the drug to the blood stream. The cannula is connected to tubing that often exits on the animal’s back and serves as the port of entry for drug delivery [40]. The animals are trained in operant boxes (Skinner boxes) to press a lever (or nose poke a hole) to obtain an IV dose of the drug. For opioids, animals readily learn the operant behavior to self-administer the drug.
Measurements of Drug Taking and Compulsive Drug Intake (Fixed-Ratio and Progressive Ratio [PR] Schedules of Reinforcement)
The operant model is versatile in terms of measurements of reward and motivation. The use of different schedules of reinforcement can provide important information on motivation for drug intake and drug reward efficacy. A fixed-ratio 1 (FR1) schedule of reinforcement is a common procedure in which every lever press performed by the animal is reinforced with a dose of the drug. Because the workload in this schedule is low and the animal easily obtains the drug, it has often been considered an operational measure of drug intake. However, in a PR schedule of reinforcement, the number of lever press that the animal needs to perform to receive the next dose of the drug increases progressively, i.e., the “price/cost” for the drug progressively increases such that the animal has to work more vigorously to obtain the next drug infusion [41, 42]. While a fixed-ratio protocol is able to measure drug intake, PR protocols are more able to measure drug reward efficacy.
Escalation of Drug Intake
Although numerous published procedures exist that produce stable levels of opioid self-administration by rodents, it has also been shown that models of extended access to opioids produces escalation of the intake over time compared with animals maintained on a limited-access schedule [43–48]. Escalation of drug intake has been suggested to model the transition from controlled drug use to compulsive drug seeking, a hallmark of drug addiction [49]. This is further supported by evidence that passive induction of an opioid-dependent state increases the rate of subsequent opioids escalation [50]. Rats that exhibit steady heroin intake show lowered intracranial self-stimulation (ICSS) thresholds associated with reward upon heroin administration, whereas escalating rats show marked elevations in ICSS thresholds, indicating a diminished impact of opioid rewarding effects [51].
Vendruscolo et al. (2011) reported that rats with 12 h access to heroin per session showed significant escalation of heroin intake across session [43]. Animals having limited (1 h) access to heroin showed relatively stable levels of heroin intake (Fig. 5.2). Food intake in the 12 h group, but not 1 h group, concomitantly decreased over sessions, with a pattern that inversely followed the escalation of heroin intake, suggesting a disruption of other natural motivated behaviors. The escalation of heroin intake in the 12 h group is likely due to the repeated cycles of intoxication and forced abstinence associated with the 12 h schedule. The negative emotional state associated with abstinence during dependence has been shown to promote drug taking [52, 53]. Confirming this hypothesis, spontaneous withdrawal signs are observed in the 12 h group, including diarrhea, vocalization upon touch, hyperalgesia/allodynia, and abnormal posture, whereas minimal (or absent) signs of withdrawal were observed in the 1 h group [43].
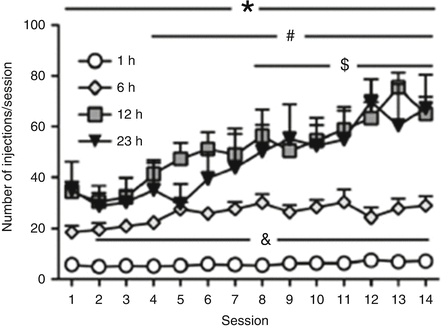
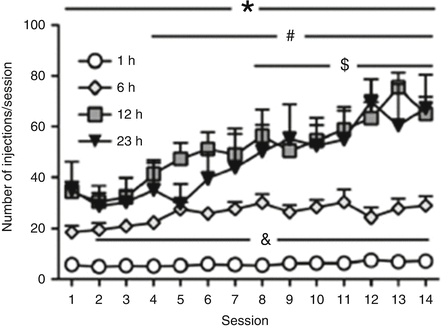
Fig. 5.2
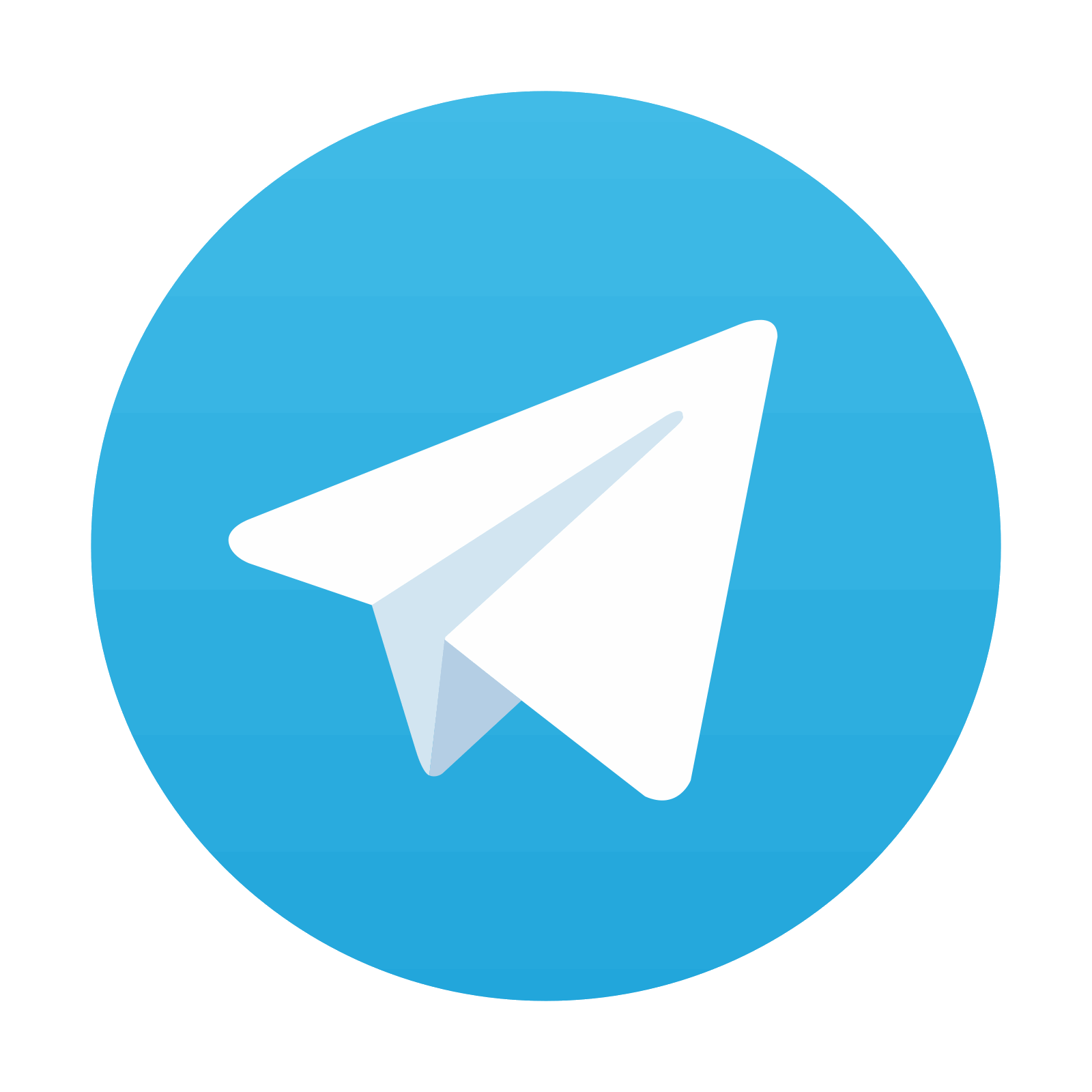
Prolonged periods of drug access result in significantly increased heroin intake over time. All animals had identical pretraining in 1 h sessions to press the active lever for heroin infusions (60 μg/kg/infusion). Animals were given continuous, unlimited access to heroin infusions for 1, 6, 12, or 23 h per day for 14 days. The 1 h access group maintained steady intake over repeated sessions. The 6 h (to a lower extent), 12 h, and 23 h access groups showed escalating patterns of intake across time. Overall, the 6, 12, and 23 h groups administered substantially more drug per session than the 1 h group. The 12 and 23 h groups self-administered significantly more heroin per session than the 6 h group, and intake in the 12 and 23 h groups had a similar magnitude. &p < 0.05, 6 h group compared with 1 h group; *p < 0.05, 12 and 23 h groups compared with 1 h group; # p < 0.05, 12 h group compared with 6 h group; and $ p < 0.05, 23 h group compared with 6 h group. Taken from Vendruscolo et al. (2010), with permission
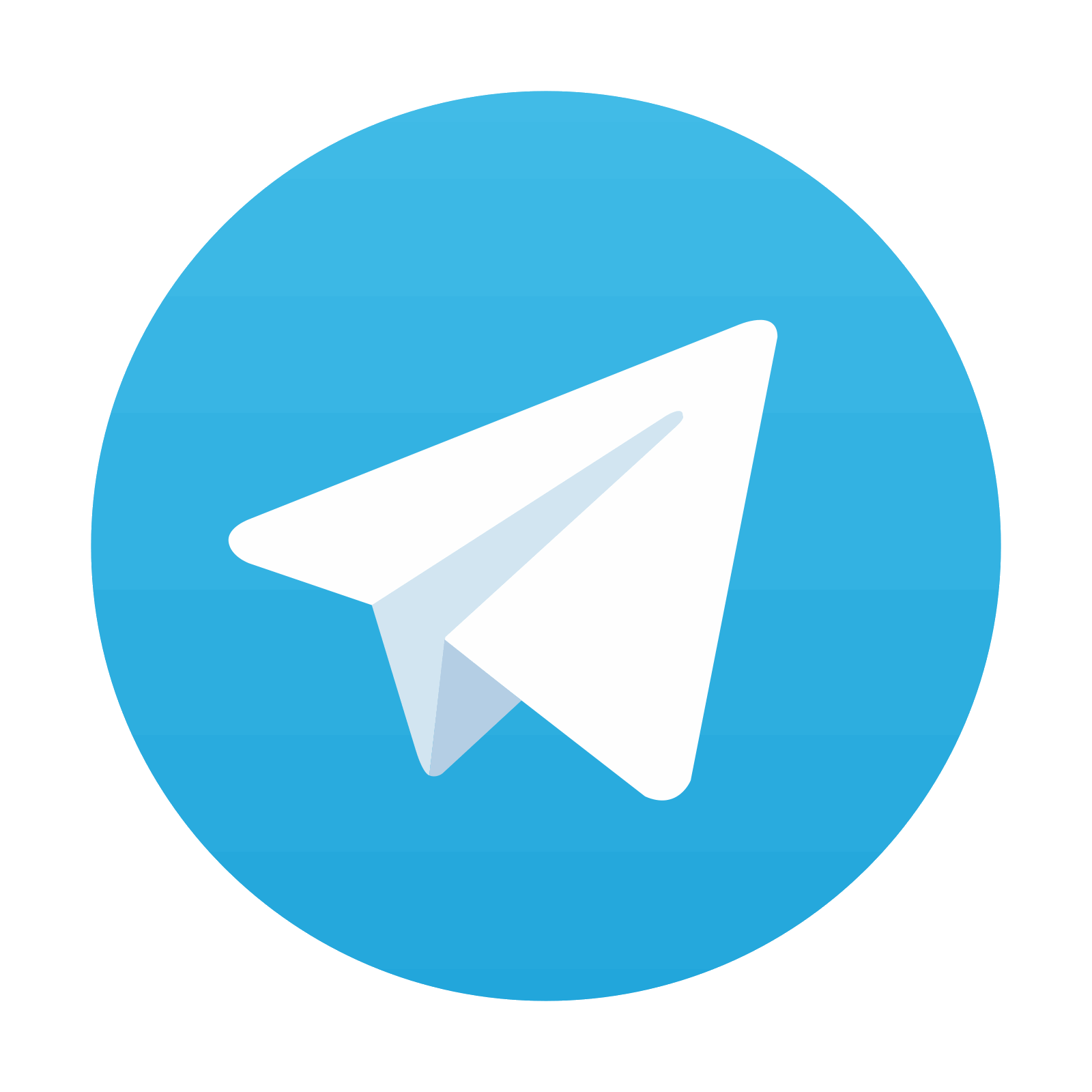
Stay updated, free articles. Join our Telegram channel
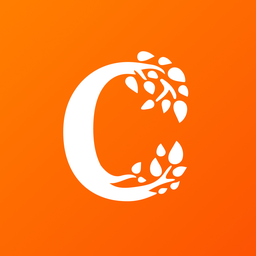
Full access? Get Clinical Tree
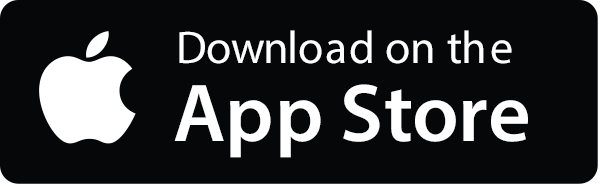
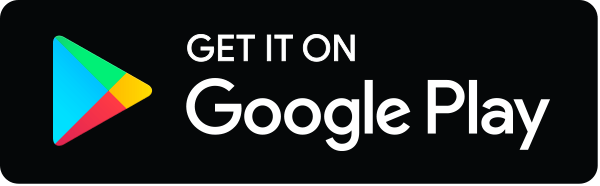
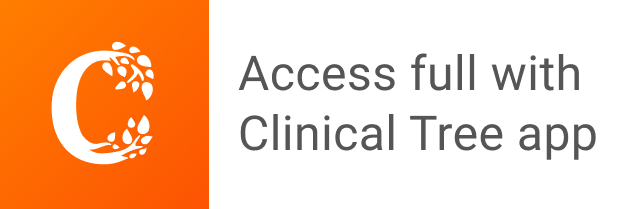