Chapter 7 Thermal effects
INTRODUCTION
Chapter 2 presented the basic scientific principles underpinning the way in which changes in temperature affect materials. This chapter examines in more detail the effects that are produced in biological materials, particularly when these are part of a functioning body.
BODY TEMPERATURE
The body is usually considered to consist of two thermal compartments: the core or central compartment, and the shell or superficial layer (Fig. 7.1).
CORE TEMPERATURE
Core temperature is approximately 37°C; arbitrary variations of ±2°C define hyper- and hypothermia, despite much larger variations in the ambient temperature in which the person is functioning (International Union of Physiological Sciences 1987). Hyperthermia can therefore be regarded as a core temperature in excess of 39°C and hypothermia a temperature below 35°C. At rest, and in a neutral environment, core temperature can be kept within a narrow band of control (±0.3°C). However, although the core temperature tends to be kept within this narrow range around 37°C, it is not a fixed entity and there are significant temperature gradients within the anatomical core. Organs such as the liver and active skeletal muscles, for example, have a higher rate of metabolic heat production than other tissues and therefore maintain a higher temperature. Similarly, there are temperature gradients within the vascular compartment perfusing both the core and the shell. In addition, core temperature varies with the body’s intrinsic diurnal temperature rhythm.
SHELL TEMPERATURE
Whereas the core temperature is relatively stable, the shell — at the interface between the body and the environment — is subject to much greater variations in temperature. Across approximately 1 cm of the body shell, from the skin surface to the superficial layer of muscle, the temperature gradient varies according to the temperature of the core and the external environment (demonstrated for the forearm in Fig. 7.2). The gradient is not uniform and changes with the thermal conductivity of the tissue layers and the rate of blood flow in the different regions. Skin temperatures differ widely over the body surface, especially in hot or cold conditions. When an individual is in a comfortable environment of, say, 24°C, the skin of the toes might be 27°C, that of the upper arms and legs 31°C and the forehead 34°C; the core is maintained at 37°C.
DIURNAL (CIRCADIAN) CORE TEMPERATURE RHYTHM
The diurnal (circadian) core temperature rhythm is one of the most stable of biological rhythms, with a well-marked intrinsic component (Fig. 7.3). Body temperature is lowest in the early morning and highest in the evening, although in a small minority of people this phase is reversed. The diurnal range of variation is usually about 0.5–1.5°C in adults, depending on other external factors, such as the effects of meals, activity, sleep and ambient temperature. Different intrinsic biological rhythms are often in phase with each other and there is evidence that when they are not, synchronization function is compromised. For example, desynchronization of the sleep–wake cycle and the core temperature cycle by continuous light exposure can bring about impairment of thermoregulatory function (Moore-Ede & Sulzman 1981). Other rhythms, which are not daily (e.g. the female menstrual cycle) also affect core temperature.
THERMAL BALANCE
For the core temperature to remain constant there needs to be a balance (equilibrium) between internal heat production, heat gain from the environment and external heat loss. This is shown pictorially in Figure 7.4 and expressed in the form of a heat balance equation (Box 7.1).
Metabolic heat production (M) is the heat produced during the work of metabolism. It can be calculated from the measurement of total body oxygen consumption. Basal metabolic rate, which occurs during complete physical and mental rest, is about 45W/m2 (i.e. watts per square metre of body surface) for an adult male of 30 years and 41W/m2 for a female of the same age. These values can be almost doubled during severe physical work and might be as high as 900W/m2 for brief periods. A small increase in M follows the eating of a meal; M is also increased by shivering.
CONTROL OF BODY TEMPERATURE
Thermoregulation is integrated by a controlling mechanism in the central nervous system (CNS) that responds to the heat of the tissues, which is detected by thermoreceptors. These receptors are sensitive to heat and cold information arising in the skin, the deep tissues and the CNS itself. They provide feedback signals to CNS structures, which are situated mainly in the hypothalamus of the brain, via a servo- or loop system (Fig. 7.5). The temperature of the blood perfusing the hypothalamus is also a major physiological drive to thermoregulation. The hypothalamus monitors ambient temperature in relation to the heat balance discussed above, and initiates appropriate physiological responses (vasodilatation and sweating in hot conditions, or vasoconstriction and shivering in cold) that counteract any deviation in the core temperature (Fig. 7.6). Apart from these involuntary responses, thermal information is transmitted by afferent nerves to regions of the brain that control endocrine functions and to the cerebral cortex, which makes individuals aware of their thermal sensations by inducing behavioural changes such as moving away from/toward heat sources, donning/removing clothing or opening/closing windows.
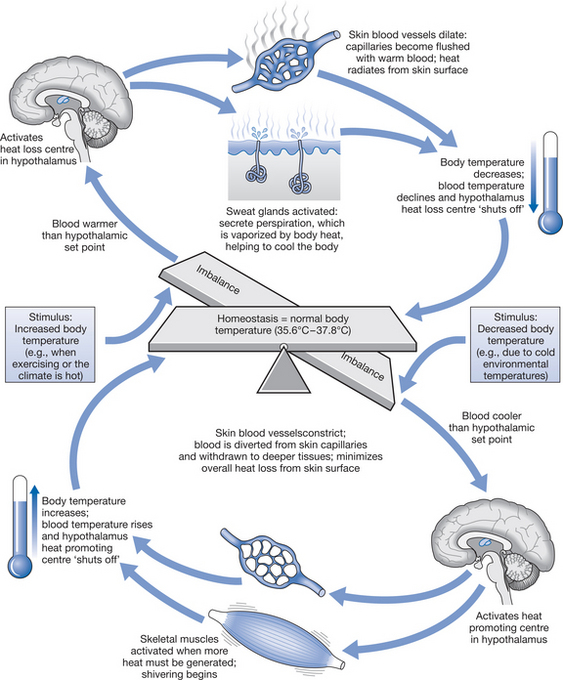
Figure 7.6 The role of the hypothalamus in thermoregulation (from Marieb 2002, with permission of Benjamin Cummings).
An essential role in processing thermal signals is ascribed to the preoptic region of the anterior hypothalamus and to a region in the posterior hypothalamus, described respectively as the ‘heat loss’ and ‘heat gain’ centres because they are considered to exert the primary control on vasodilatation/sweating in the heat and on vasoconstriction/shivering in a cold environment. The integration of incoming and outgoing information, and the ‘set-point’ from which the hypothalamic centres operate, is the basis on which present views of thermoregulatory control are constructed (Collins 1992, Hensel 1981).
PHYSIOLOGICAL EFFECTS OF THERMAL CHANGES
PHYSIOLOGICAL EFFECTS OF COLD
Blood flow
A further effect is seen, for example, in the hand. Immersion of the hands in water at 0–12°C at first causes the expected vasoconstriction, this is followed after a delay of 5 minutes or more by a marked vasodilatation. This is then interrupted by another burst of vasoconstriction and subsequent waves of increased and decreased local blood flow. This phenomenon is known as cold-induced vasodilatation (CIVD) and demonstrates a hunting reaction of the vessels, which can be measured simply by thermocouple readings on the cooled skin (Fig. 7.7). CIVD is most likely to be due to the direct effect of low temperature causing paralysis of smooth muscle contraction in the blood vessels (Keatinge 1978). The reaction — which does not only occur in the hand — might provide protection to tissues from damage caused by prolonged cooling and relative ischaemia. There is a marked difference in the appearance of the skin erythema due to CIVD compared to that produced by skin heating. In CIVD, the skin has a brighter red colour owing to the presence of more oxyhaemoglobin and less reduced haemoglobin in blood. The reason for this is that at low temperatures there is a shift in the oxygen dissociation curve so that the blood tends to hold on to its oxygen, with oxyhaemoglobin dissociating less readily.
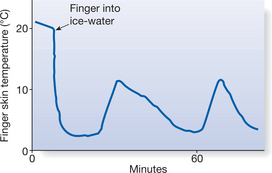
Figure 7.7 Cold vasodilatation in the finger immersed in ice-water, measured by skin temperature changes.
Muscle blood flow is not much influenced by thermal reflexes but is determined largely by local muscle metabolic rate. A striking feature of attempts at muscle cooling is the prolonged period taken to reduce intramuscular temperatures: muscles are insulated from temperature changes at the skin surface by a layer of subcutaneous tissue (Jutte et al 2001, Otte et al 2002).
Collagen
Although most studies examining stretch in collagen focus on the effects of higher temperatures (e.g. Rigby et al 1959, 1964, Warren et al 1971) with little below 25°C, it is reasonable to expect that collagen tends to become stiffer when cooled. The degree to which this happens, and at what temperatures, is not clear. Experience tells us that very cold hands can feel ‘stiff’ and individuals with rheumatoid arthritis often complain of an increase in stiffness as temperatures are reduced. However, whether this effect is purely the result of changes in collagen requires much more work.
Pain relief
Cold can be used as a counterirritant, and it has been suggested that such responses might be explained on the basis of the pain gate theory (see Chapter 6). Effects can also be mediated through the effects of the morphine receptors in the CNS, and the role of the enkephalins and endorphins (Doubell et al 1999, Fields & Basbaum 1999). It has been demonstrated that peripheral nerve conduction is slowed by cold (Lee et al 1978), finally ceasing altogether. Fibres vary in their sensitivity according to their diameter and whether they are myelinated, and animal studies have demonstrated that the small-diameter myelinated fibres (i.e. Aδ fibres), which conduct pain, are most responsive to cold. Although it would be unwise to extrapolate all these findings wholly to humans, this combined evidence suggests that effects on nerve fibres and free nerve endings lead to a reduction in pain.
Pain can sometimes be due to particular tissue irritants. For example, a number of studies have suggested that patients with arthritis experience pain relief as a result of the adverse effects of cooling on the activity of destructive enzymes within the joints (Harris & McCroskery 1974, Pegg et al 1969).
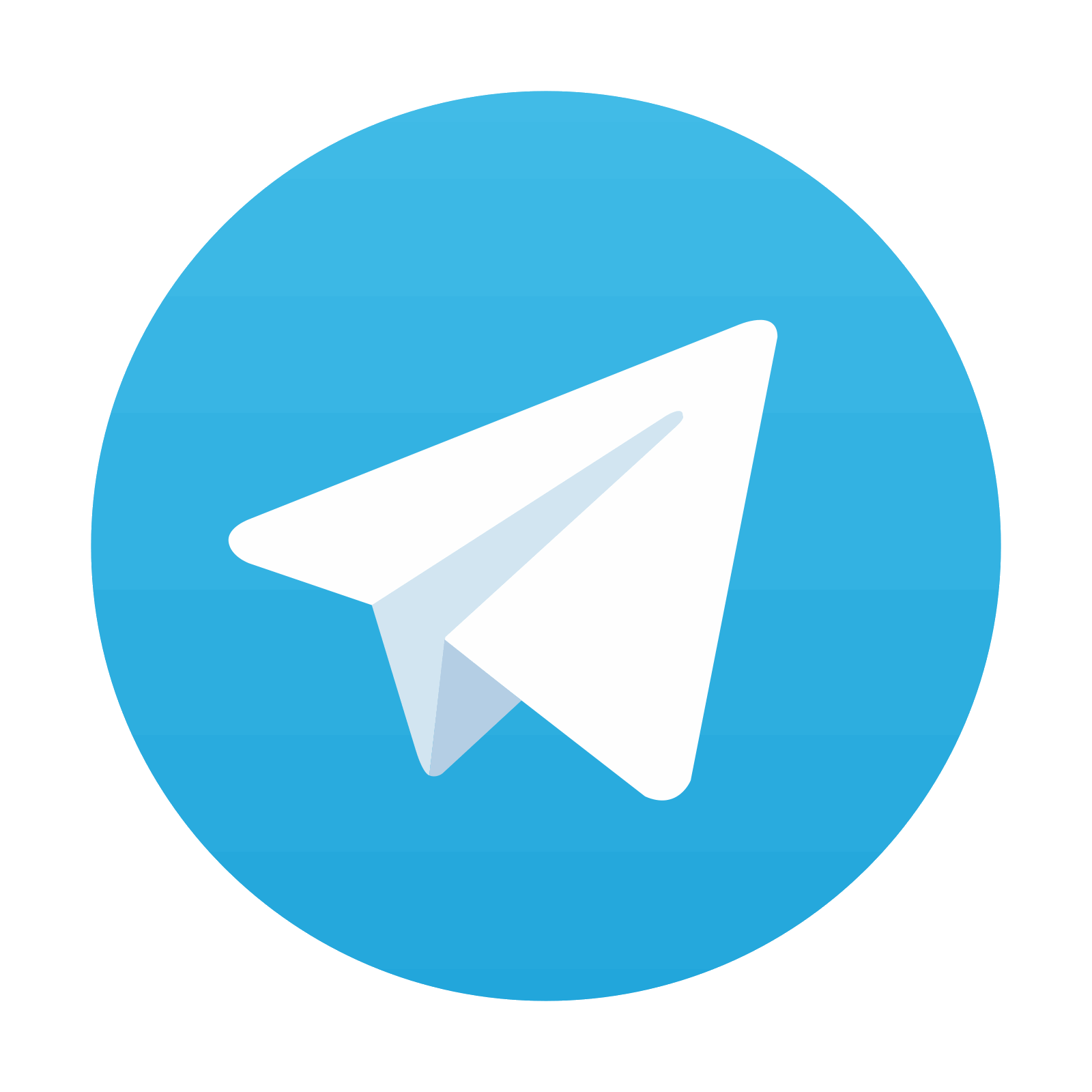
Stay updated, free articles. Join our Telegram channel
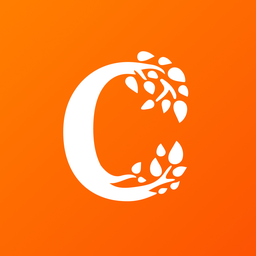
Full access? Get Clinical Tree
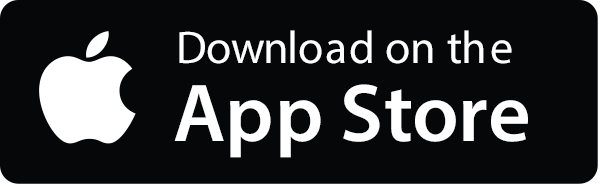
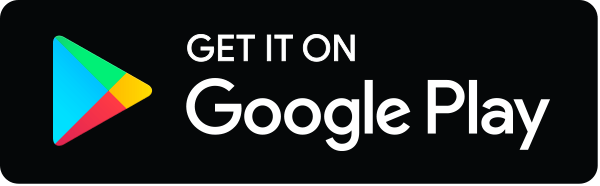