Direct electrical stimulation of the brain is an increasingly popular means of treating refractory epilepsy. Although there has been moderate success in human trials, the rate of seizure freedom does not yet compare favorably to resective surgery. It therefore remains critical to advance experimental investigations aimed toward understanding brain stimulation and its utility. This article introduces the concepts necessary for understanding these experimental studies, describing recording and stimulation technology, animal models of epilepsy, and various subcortical targets of stimulation. Bidirectional and closed-loop device technologies are also highlighted, along with the challenges presented by their experimental use.
Direct electrical stimulation of the brain is an increasingly popular means of treating refractory epilepsy. Although there has been moderate success in human trials, the rate of seizure freedom does not yet compare favorably to resective surgery. It therefore remains critical to advance experimental investigations aimed toward understanding brain stimulation and its utility. This article introduces the concepts necessary for understanding these experimental studies, describing recording and stimulation technology, animal models of epilepsy, and the various subcortical targets of stimulation that have been investigated. Because of the continued interest in bidirectional and closed-loop devices, these technologies are also highlighted, along with the challenges presented by their experimental use.
Electrode recording
Types of Signals
Depending on the properties of the recording electronics (electrode material, size, position, sampling rate of the analog-to-digital converters, and so forth), signals ranging from individual action potentials to the electrocorticogram can be recorded, with each type of signal providing a different scale of information about brain physiology.
Single units
When a neuron generates an action potential (AP), the current across its cell membrane generates a complex electric field. An electrode placed in this external electric field will detect this activity, referred to as a spike . Recording such spiking activity from individual neurons is known as single unit recording. Unlike intracellularly recorded APs, which are commonly recorded using patch-clamp techniques, extracellular spikes vary markedly in morphology and even polarity, and are dependent on the geometry of the cell and the location of the recording electrode. Although these variations may be of interest, in that they may indicate location of the recording electrode in well-defined neural tissue, most investigations instead examine evoked spikes or characteristics of spike trains. Microelectrodes with a tip diameter on the order of micrometers and geometric surface area less than 2000 to 4000 μm 2 are commonly used for recording the extracellular activity of single neurons. The extracellularly recorded action potential is typically less than 100 μV, about 2 orders of magnitude lower than that of a corresponding intracellular recording.
During single-unit recordings, the largest component of signal noise arises from the multitude of undifferentiated background action potentials and synaptic potentials (neural noise). However, electrode impedance also contributes and is known as thermal noise, with higher impedance electrodes consequently demonstrating more noise and a lower signal-to-noise ratio. In addition, high electrode impedance in combination with the distributed capacitance between the electrode and the recording amplifier will reduce the electrodes’ high-frequency response. One method of improving the signal-to-noise ratio is to reduce electrode impedance without increasing the surface extent of the electrodes. This can be achieved either through sputtering or electroplating the tip with a biocompatible material that reduces the impedance without increasing the surface extent of the electrodes.
Multiunit activity
If activity from more than one single neuron is detected by a microelectrode, the resulting polyneuronal activity is often referred to as multiunit activity (MUA). Spike-sorting methods can then be used to putatively identify each recorded waveform as originating from a particular cell. However, another definition of MUA relies on isolating the “neural noise” described previously. If the electrical activity above a certain frequency is isolated (eg, >300 Hz), the power of those high frequencies can be used as a surrogate for large-scale spiking activity from multiple neurons. This high-pass signal is then typically rectified and low-pass filtered to generate a time-varying estimate of MUA.
Local field potentials
The local field potential (LFP) is the low-frequency (arbitrarily <300 Hz) summed synaptic activity recorded extracellularly from a population of neurons. It can be isolated from microelectrodes or macroelectrodes. Recent studies suggest that the LFP signal originates within a range of 250 μm from the recording electrode, smaller than the previously reported range of 400 μm to a few millimeters.
LFP recordings enable the visualization of prominent brain oscillations (eg, alpha, beta, theta, gamma, delta) when electrodes are placed in appropriate regions. There is some debate about whether the various frequency components of the LFP have unique corresponding spatial footprints. For example, it has been proposed that the higher frequencies (in the gamma range, around 25–90 Hz) may carry information that is more local than the lower frequencies, perhaps as a result of the capacitive properties of neural tissue.
Electrocorticography
Electrocorticography (ECoG) uses macroelectrodes (∼5 mm in diameter) to record directly from the brain, either superficially or at depth. Although, as compared with electroencephalography (EEG), ECoG is invasive, it enables higher spatial resolution (ie, tenths of millimeters vs centimeters), broader bandwidth (ie, 0–200 Hz vs 0–40 Hz), and higher amplitude (ie, 50–100 μV maximum vs 10–20 μV). Surface ECoG does not require electrodes to penetrate the cortex, and thus may have greater long-term stability and safety compared with single-unit penetrating microelectrodes ; however, it lacks the spatial resolution of implanted microelectrode and macroelectrode recordings.
Electroencephalography
EEG refers to electrical recordings made with macro electrodes positioned noninvasively on the subject’s scalp (but could include other approaches, eg, epidural peg electrodes). Standard montages are used (eg, the international 10–20 system), but are often supplanted by extra electrodes. EEG is most commonly used in the diagnosis and classification of epilepsy, with its main advantages being that it is noninvasive and inexpensive. However, EEG does have several limitations. Large areas of the cortex need to be activated synchronously to generate enough potential to be recorded extracranially. Propagation of electrical activity along physiologic pathways in extracellular spaces may give a misleading impression as to the source of the electrical activity. Furthermore, EEG suffers from poor spatial resolution, confounding seizure foci localization. Nevertheless, EEG continues to play a central role in the diagnosis of epilepsy and other neurologic disorders, and has been used extensively in conjunction with other diagnostic techniques.
Types of Electrodes
According to Merrill and colleagues, the ideal material for building electrodes should satisfy 6 requirements. It should (1) be biologically compatible, (2) maintain its mechanical integrity during the surgical procedure, (3) have sufficient charge storage capacity, (4) not undergo electrochemical reactions that produce toxic products, (5) not undergo Faradaic corrosion reactions, and (6) be stable for the duration of the implant. Criterion (5) depends highly on the duration of implantation—electrodes used in acute studies are unlikely to fail because of corrosion. Based on their size, electrodes can be broadly classified into 2 categories: microelectrodes and macroelectrodes.
Microelectrodes
Microelectrodes are small-diameter (<50 μm) electrodes, typically used in the recording of single-unit activity. Depending on the material and diameter, their impedances range from tens of kΩ to more than tens of MΩ. In addition to recording, microelectrodes can be used to deliver stimulation. In a direct application of Ohm’s law, the higher the impedance of the microelectrode, the higher the required voltage to deliver the same amount of current. Thus, microelectrode impedance plays a major role in determining the maximum stimulation potential that may be applied to the electrode without causing undesirable breakdown of water molecules into hydrogen and oxygen gas. This potential limit is known as the “water window,” and with high impedance electrodes, care should be taken to ensure the stimulation potential does not exceed an acceptable range. One way of circumventing this problem is to reduce the impedance of microelectrodes while maintaining their small surface extent, for example by electroplating their tips with a biocompatible material. The material deposited with electroplating, however, can sometimes be shed during long recordings, limiting the use of electroplating in chronic recordings. More recently it has been shown by our group that sonicoplating—electroplating under ultrasonic agitation—can significantly improve the durability of plating. Lowering microelectrode impedance will produce additional advantages, such as reduced thermal noise, which increases as a function of the electrode impedance, and smaller stimulation artifacts. Reduction of stimulation artifact is particularly important for closed-loop applications, which are discussed later in this article.
Metal microelectrodes
The performance of metal microelectrodes for neural recording and stimulation depends on their geometric, electrical, and mechanical properties. The most frequently used metals for manufacturing microelectrodes include stainless steel, platinum, iridium, tungsten, titanium, nickel-chromium, and their alloys. Copper and silver are known to cause tissue necrosis and hence are not often used. Electrodes are often covered in an insulating material—such as varnish, epoxy, parylene, glass, Teflon, or polyimide—to within a few micrometers of their tip, which is left exposed to record the highly localized extracellular potentials induced by currents in one or a few of the closest neurons. They may also be used to deliver highly localized stimulation.
Tetrodes can be made by twisting 4 microwires around each other and applying sufficient heat to bind them together by melting their insulation. The 4 microelectrode tips are then within a micrometer from each other, and consequently have a high probability of picking up APs simultaneously from the same neurons. The shape of the APs picked up by the 4 electrodes, although different (dependent on their location with respect to the firing neuron) is also very reproducible (they record the same shape of activity whenever that particular neuron fires), making spike sorting simple and reliable, using a process akin to triangulation to identify particular cells. The tetrode configuration additionally provides stability to the microwires during implantation. Another common practice is to arrange multiple microwires onto a single shaft (microwire arrays) for recording and/or stimulating multiple single units at the same time. TDT (Achua, FL, USA), Neuronexus Technologies Inc (Ann Arbor, MI, USA), and Plexon (Dallas, TX, USA) are among the vendors who sell such microwire arrays for use in animal studies. More recently, Bartels and colleagues, have designed neurotrophic electrodes for increasing the longevity and stability of recorded signals. Their design induces neurite growth into a glass cone that covers the tip of gold microelectrodes insulated with Teflon, anchoring the glass tip to the neuropil.
Other types of microelectrodes
Silicone-based microelectrode arrays are gaining popularity as an alternative to microwire arrays. The most significant advantage of the silicon-based microelectrode technology is that it enables the monolithic integration of electronics, such as amplifiers, filters, and multiplexers, into the probe structure. These active microelectrodes improve the quality of recorded signals over passive microelectrode arrays (without integration of electronics).
PDMS (polydimethylsiloxane)-based microelectrodes are deformable, enabling conformation to the structure from which they are recording and/or stimulating. Such flexible designs hold great promise for recording from structures that are otherwise inaccessible to the rigid metal/silicone-based microelectrodes, although they are not yet available for use in human subjects ( Fig. 1 ).

Macroelectrodes
Macroelectrodes have dimensions on the order of a few millimeters (typically 1–5 mm), with a corresponding surface area around 1–25 mm 2 , and impedance in the range of 500–1000 Ω. Consequently, they are primarily used to record LFP, ECoG, and EEG signals.
Depth macroelectrodes
Depth electrodes are widely used in epileptic patients to delineate seizure foci. Typical depth electrodes consist of 4 or more 1-mm contacts arranged linearly on a single shaft, separated by a distance of approximately 10 mm. In addition to recording, similar electrodes are also used for stimulation (eg, deep brain stimulation in Parkinson disease and epilepsy). Several groups have investigated the impact of electrode geometry on recorded and stimulated volumes of brain tissue, topography, and so forth. Recently, hybrid depth electrodes, consisting of both macroelectrodes and microwires arranged on the same shaft, have been used for simultaneous recording of LFPs and single-unit activity. Image guidance techniques are used to ensure proper placement of depth electrodes.
Surface macroelectrodes
ECoG recordings often make use of grid and strip subdural surface electrodes. In many cases subdural electrodes are used in combination with depth electrodes to delineate seizure foci. Like hybrid depth electrodes, hybrid surface electrodes—with microelectrodes interspersed between macroelectrodes—are becoming popular owing to their increased spatial resolution, and for research purposes.
Stimulation
Mechanisms of Stimulation
Little has changed in the 35 years since Ranck observed that “despite the extensive use of electrical stimulation of the central nervous system, both clinically and experimentally, there has been little concern with what cells or parts of cells are stimulated.” To this day, the mechanism of action of electrical stimulation on the nervous system remains poorly understood. What has become clear, however, is that many parameters of stimulation (electrode configuration, pulse width, frequency, and so forth), as well as the architectural organization of the stimulated tissue, are critical in determining its impact.
Ranck noted that thresholds were lowest in myelinated axons, and progressively increased in unmyelinated axons, dendrites, and cell bodies. This point was further substantiated in a series of elegant experiments by Histed and colleagues. Using simultaneous low-current 250 Hz electrical microstimulation and 2-photon calcium imaging in mouse and cat visual cortex, the investigators were able to examine a large population of cells, free of confounding stimulus artifacts, to identify the population and location of neurons activated by cortical microstimulation. Their work revealed a sparse and distributed population of activated neurons that possessed several additional intriguing qualities. Rather than activating neurons whose cell bodies were closest to the electrode tip, cells were sparsely activated in a region surrounding the electrode, in some cases up to 4 mm away. Increasing the stimulating current amplitude increased the number of cells activated within this area, but did not activate those farther away. In fact, a large percentage of the neurons identified failed to be activated, even as their immediate neighbors were. These experiments suggest microstimulation is primarily acting on neural elements (hypothesized to be axons) as opposed to cell bodies. If axons are indeed the primary target, it is further unclear what regions are most susceptible to electrical stimulation, with the neuron initial segment and nodes of Ranvier being variably implicated.
Little more is known as to whether electrical stimulation primarily acts in an inhibitory or excitatory manner. One of the more accepted theories suggests that neurons adjacent to stimulating electrodes undergo long-term inactivation as a result of stimulation. This may be based on the similarities in clinical outcome between surgical ablation and deep brain stimulation ; however, activation of efferent neurons, even in the context of inhibited cell bodies, has been demonstrated in several reports. Indeed, even Ranck noted that at high currents, elements nearby the electrode would fail to respond, whereas those in a surrounding sphere were activated. Consequently, a single stimulus could produce variable regions of activation and inhibition. The local suppression of APs and bursting activity has been noted by other groups as well: Lian and colleagues found that 50-Hz sinusoidal current injected locally through a monopolar electrode could effectively block activity in a region close to the stimulation site, but was unable to completely suppress activity remotely. The mechanism underlying this suppression was posited to be through a local depolarization block from a large increase in extracellular potassium, a well-described phenomenon associated with high-frequency alternating current (AC) stimulation. Indeed, whereas electrical stimulation itself may be activating neural elements, the network effects may actually be inhibitory because of changes in electrochemical gradients, neurotransmitter depletion, or signal obfuscation.
These observations have served to reinforce early indications that stimulation parameters are crucial for determining clinical outcomes. Despite this, human trials are largely based on empiric evidence and case reports. Ideally, clinicians would tune treatment based on knowledge of the various interdependent properties of electrical stimulation, so as to maximize effectiveness and reduce side effects. Although there is much work to be done before the latter can become standard, current understanding should prove useful in improving clinical efficacy and research methods.
Targeting
Although gross targeting of stimuli to anatomically relevant tissue plays an important role in therapeutic outcome, local cellular cytoarchitecture has been implicated as well. High-frequency deep brain stimulation (DBS) for parkinsonian tremor has primarily been targeted to neurons of the subthalamic nucleus, but it is unclear whether or not the effect is primarily mediated through the local neuron bodies or through fibers of passage. Voges and colleagues found the best improvements involved electrodes projecting onto fiber tracts located close to the subthalamic nucleus (STN), and recent optogenetic investigations have implicated fibers of passage to the nuclei itself.
Anatomic targets may be better reflected in orthodromic and antidromic projections, as opposed to their local soma. Furthermore, orientation within these regions has the potential to be highly influential. Ranck 32 determined that more current was necessary to generate the same results if monopolar electrodes were positioned in such a way that current flow was across the axon (transverse) rather than parallel to it (longitudinal). Indeed, it was only when anodic fields were applied across the dendritic-somatic axis in slice preparations of epileptic hippocampus that seizures could be effectively suppressed. Applying the field perpendicularly to this axis, however, was unable to affect activity even at high field values. Interestingly, Lian and colleagues found that orientation of a monopolar electrode along the dendritic-somatic axis during AC stimulation (sinusoidal, 50 Hz, 0 direct current [DC] offset) had no effect on suppression, whereas DC stimulation was highly orientation-dependent. Higher-frequency stimulation (>50 Hz) may indeed be orientation independent, despite the initial findings of Ranck. 32 This may be consequent to blockade of voltage-gated currents or accumulation of extracellular potassium or other biochemicals (eg, adenosine ), which could occur independently from the direction of current flow.
Frequency
Frequency of stimulation, as implicated previously, can have an important influence as well. Aside from matters of orientation, different network-wide impacts have been implicated for high-frequency (roughly >50 Hz), low-frequency (<50 Hz), and DC stimulation. High-frequency stimulation can induce peripheral nerve blocks, and in the subthalamic nucleus was seen to inhibit somatic neuronal activity and axonal conduction in hippocampal preparations in vitro and in vivo. High-frequency stimulation may thus act, in part, through a conduction blockade of pathologic activity, hence preventing spread and/or synchronization. However, effects on efferent axons independent of effects on the cell soma must be considered as well.
Low-frequency stimulation (LFS) is presumed to be excitatory in nature, and was seen to reduce seizure frequency in human patients with epilepsy, as well as in several animal models of epilepsy. LFS could be inhibiting, however, as it may induce long-term depression through the alteration of synaptic weights. It is possible that LFS activates axons, and that this activation, being asynchronous with synaptic input, generates LTD and reduces the activity of the overexcited cell. Further research is necessary to determine the exact mechanisms underlying LFS and its impact on synaptic weighting. Preliminary evidence suggests that LFS may restore normal network responses.
DC electric fields can also be applied to brain tissue to suppress spontaneous epileptiform activity. These fields are often created in vitro using parallel wires or plates. Anodic fields generate higher potentials at the cell soma vis-à-vis dendrites, resulting in positive current flow into the soma and out through the dendrites. This hyperpolarizes the soma, preventing excitation to threshold and conduction of epileptiform activity. Even with the obvious difficulties with in vivo applications, there are additional drawbacks to the technique. Unbalanced charge deposition results in erosion and electrochemical tissue damage. Further, suppression produces rebound excitation on removal. These limitations make the application of such fields a challenge in human patients.
Polarity
Electrode configurations tend to be divided into monopolar and multipolar varieties, the most common of which is bipolar. All configurations (even so-called monopolar) consist of an anode and a cathode, with current flowing from the cathode toward the anode. In monopolar electrodes, the cathode is distant from the anode. For example, DBS stimulators will often use the pulse generator case as the anode. Multipolar configurations have the anode and cathode in relative proximity, such as between neighboring contacts. Monopolar configurations thus create broader extracellular current spread than multipolar arrangements, and consequently, bipolar configurations are less likely to generate unwanted side effects. Multipolar configurations allow for greater control of current distribution, enabling more precise targeting.
Waveform
Most current clinical neural stimulators make use of rectangular, charge-balanced waveforms. Much of this adherence may be based on historical precedent, although charge-balancing helps reduce electrode erosion and electrochemical tissue damage. Aside from potential energy savings and prolongation of stimulator battery lifetime, alternative stimulus waveforms have the potential to target different regions in nervous tissue, and may be the difference between an inhibitory or an excitatory response. More research must be performed to support the implications of modeling studies.
Amplitude
It has long been assumed that increasing current amplitude results in a larger sphere of activated neuron bodies around the electrode contact. However, as described earlier, stimulation results in an activation of cells in a distinct region determined by the local neuroanatomy. Higher amplitudes activate a greater number of fibers, thus increasing the number of cell bodies activated. This does not significantly increase the distance at which activation occurs, as it is determined by the anatomically-defined axonal projections of the neurons, rather than the soma’s distance from the electrode tip. Consequently, although higher-amplitude stimulation recruits a larger proportion of neurons, which neurons are influenced, and at what distance from the tip, seems to be determined by the local cytoarchitecture.
Current-Controlled Versus Voltage-Controlled Stimulation
In current-controlled stimulation, which is more commonly used experimentally, current is kept constant throughout the pulse period, whereas voltage varies as a function of impedance. Lempka and colleagues have shown that current-controlled stimulation may be more effective in maintaining constant voltage distribution, as the impedance of the electrode-electrolyte interface changes drastically with time. In the voltage-controlled stimulation, the voltage is kept constant while the current is allowed to vary. This method is easier to implement technically despite its lack of popularity. It is noted that some studies have shown greater efficacy of voltage-over current-controlled pulses in eliciting neural responses.
Stimulation
Mechanisms of Stimulation
Little has changed in the 35 years since Ranck observed that “despite the extensive use of electrical stimulation of the central nervous system, both clinically and experimentally, there has been little concern with what cells or parts of cells are stimulated.” To this day, the mechanism of action of electrical stimulation on the nervous system remains poorly understood. What has become clear, however, is that many parameters of stimulation (electrode configuration, pulse width, frequency, and so forth), as well as the architectural organization of the stimulated tissue, are critical in determining its impact.
Ranck noted that thresholds were lowest in myelinated axons, and progressively increased in unmyelinated axons, dendrites, and cell bodies. This point was further substantiated in a series of elegant experiments by Histed and colleagues. Using simultaneous low-current 250 Hz electrical microstimulation and 2-photon calcium imaging in mouse and cat visual cortex, the investigators were able to examine a large population of cells, free of confounding stimulus artifacts, to identify the population and location of neurons activated by cortical microstimulation. Their work revealed a sparse and distributed population of activated neurons that possessed several additional intriguing qualities. Rather than activating neurons whose cell bodies were closest to the electrode tip, cells were sparsely activated in a region surrounding the electrode, in some cases up to 4 mm away. Increasing the stimulating current amplitude increased the number of cells activated within this area, but did not activate those farther away. In fact, a large percentage of the neurons identified failed to be activated, even as their immediate neighbors were. These experiments suggest microstimulation is primarily acting on neural elements (hypothesized to be axons) as opposed to cell bodies. If axons are indeed the primary target, it is further unclear what regions are most susceptible to electrical stimulation, with the neuron initial segment and nodes of Ranvier being variably implicated.
Little more is known as to whether electrical stimulation primarily acts in an inhibitory or excitatory manner. One of the more accepted theories suggests that neurons adjacent to stimulating electrodes undergo long-term inactivation as a result of stimulation. This may be based on the similarities in clinical outcome between surgical ablation and deep brain stimulation ; however, activation of efferent neurons, even in the context of inhibited cell bodies, has been demonstrated in several reports. Indeed, even Ranck noted that at high currents, elements nearby the electrode would fail to respond, whereas those in a surrounding sphere were activated. Consequently, a single stimulus could produce variable regions of activation and inhibition. The local suppression of APs and bursting activity has been noted by other groups as well: Lian and colleagues found that 50-Hz sinusoidal current injected locally through a monopolar electrode could effectively block activity in a region close to the stimulation site, but was unable to completely suppress activity remotely. The mechanism underlying this suppression was posited to be through a local depolarization block from a large increase in extracellular potassium, a well-described phenomenon associated with high-frequency alternating current (AC) stimulation. Indeed, whereas electrical stimulation itself may be activating neural elements, the network effects may actually be inhibitory because of changes in electrochemical gradients, neurotransmitter depletion, or signal obfuscation.
These observations have served to reinforce early indications that stimulation parameters are crucial for determining clinical outcomes. Despite this, human trials are largely based on empiric evidence and case reports. Ideally, clinicians would tune treatment based on knowledge of the various interdependent properties of electrical stimulation, so as to maximize effectiveness and reduce side effects. Although there is much work to be done before the latter can become standard, current understanding should prove useful in improving clinical efficacy and research methods.
Targeting
Although gross targeting of stimuli to anatomically relevant tissue plays an important role in therapeutic outcome, local cellular cytoarchitecture has been implicated as well. High-frequency deep brain stimulation (DBS) for parkinsonian tremor has primarily been targeted to neurons of the subthalamic nucleus, but it is unclear whether or not the effect is primarily mediated through the local neuron bodies or through fibers of passage. Voges and colleagues found the best improvements involved electrodes projecting onto fiber tracts located close to the subthalamic nucleus (STN), and recent optogenetic investigations have implicated fibers of passage to the nuclei itself.
Anatomic targets may be better reflected in orthodromic and antidromic projections, as opposed to their local soma. Furthermore, orientation within these regions has the potential to be highly influential. Ranck 32 determined that more current was necessary to generate the same results if monopolar electrodes were positioned in such a way that current flow was across the axon (transverse) rather than parallel to it (longitudinal). Indeed, it was only when anodic fields were applied across the dendritic-somatic axis in slice preparations of epileptic hippocampus that seizures could be effectively suppressed. Applying the field perpendicularly to this axis, however, was unable to affect activity even at high field values. Interestingly, Lian and colleagues found that orientation of a monopolar electrode along the dendritic-somatic axis during AC stimulation (sinusoidal, 50 Hz, 0 direct current [DC] offset) had no effect on suppression, whereas DC stimulation was highly orientation-dependent. Higher-frequency stimulation (>50 Hz) may indeed be orientation independent, despite the initial findings of Ranck. 32 This may be consequent to blockade of voltage-gated currents or accumulation of extracellular potassium or other biochemicals (eg, adenosine ), which could occur independently from the direction of current flow.
Frequency
Frequency of stimulation, as implicated previously, can have an important influence as well. Aside from matters of orientation, different network-wide impacts have been implicated for high-frequency (roughly >50 Hz), low-frequency (<50 Hz), and DC stimulation. High-frequency stimulation can induce peripheral nerve blocks, and in the subthalamic nucleus was seen to inhibit somatic neuronal activity and axonal conduction in hippocampal preparations in vitro and in vivo. High-frequency stimulation may thus act, in part, through a conduction blockade of pathologic activity, hence preventing spread and/or synchronization. However, effects on efferent axons independent of effects on the cell soma must be considered as well.
Low-frequency stimulation (LFS) is presumed to be excitatory in nature, and was seen to reduce seizure frequency in human patients with epilepsy, as well as in several animal models of epilepsy. LFS could be inhibiting, however, as it may induce long-term depression through the alteration of synaptic weights. It is possible that LFS activates axons, and that this activation, being asynchronous with synaptic input, generates LTD and reduces the activity of the overexcited cell. Further research is necessary to determine the exact mechanisms underlying LFS and its impact on synaptic weighting. Preliminary evidence suggests that LFS may restore normal network responses.
DC electric fields can also be applied to brain tissue to suppress spontaneous epileptiform activity. These fields are often created in vitro using parallel wires or plates. Anodic fields generate higher potentials at the cell soma vis-à-vis dendrites, resulting in positive current flow into the soma and out through the dendrites. This hyperpolarizes the soma, preventing excitation to threshold and conduction of epileptiform activity. Even with the obvious difficulties with in vivo applications, there are additional drawbacks to the technique. Unbalanced charge deposition results in erosion and electrochemical tissue damage. Further, suppression produces rebound excitation on removal. These limitations make the application of such fields a challenge in human patients.
Polarity
Electrode configurations tend to be divided into monopolar and multipolar varieties, the most common of which is bipolar. All configurations (even so-called monopolar) consist of an anode and a cathode, with current flowing from the cathode toward the anode. In monopolar electrodes, the cathode is distant from the anode. For example, DBS stimulators will often use the pulse generator case as the anode. Multipolar configurations have the anode and cathode in relative proximity, such as between neighboring contacts. Monopolar configurations thus create broader extracellular current spread than multipolar arrangements, and consequently, bipolar configurations are less likely to generate unwanted side effects. Multipolar configurations allow for greater control of current distribution, enabling more precise targeting.
Waveform
Most current clinical neural stimulators make use of rectangular, charge-balanced waveforms. Much of this adherence may be based on historical precedent, although charge-balancing helps reduce electrode erosion and electrochemical tissue damage. Aside from potential energy savings and prolongation of stimulator battery lifetime, alternative stimulus waveforms have the potential to target different regions in nervous tissue, and may be the difference between an inhibitory or an excitatory response. More research must be performed to support the implications of modeling studies.
Amplitude
It has long been assumed that increasing current amplitude results in a larger sphere of activated neuron bodies around the electrode contact. However, as described earlier, stimulation results in an activation of cells in a distinct region determined by the local neuroanatomy. Higher amplitudes activate a greater number of fibers, thus increasing the number of cell bodies activated. This does not significantly increase the distance at which activation occurs, as it is determined by the anatomically-defined axonal projections of the neurons, rather than the soma’s distance from the electrode tip. Consequently, although higher-amplitude stimulation recruits a larger proportion of neurons, which neurons are influenced, and at what distance from the tip, seems to be determined by the local cytoarchitecture.
Current-Controlled Versus Voltage-Controlled Stimulation
In current-controlled stimulation, which is more commonly used experimentally, current is kept constant throughout the pulse period, whereas voltage varies as a function of impedance. Lempka and colleagues have shown that current-controlled stimulation may be more effective in maintaining constant voltage distribution, as the impedance of the electrode-electrolyte interface changes drastically with time. In the voltage-controlled stimulation, the voltage is kept constant while the current is allowed to vary. This method is easier to implement technically despite its lack of popularity. It is noted that some studies have shown greater efficacy of voltage-over current-controlled pulses in eliciting neural responses.
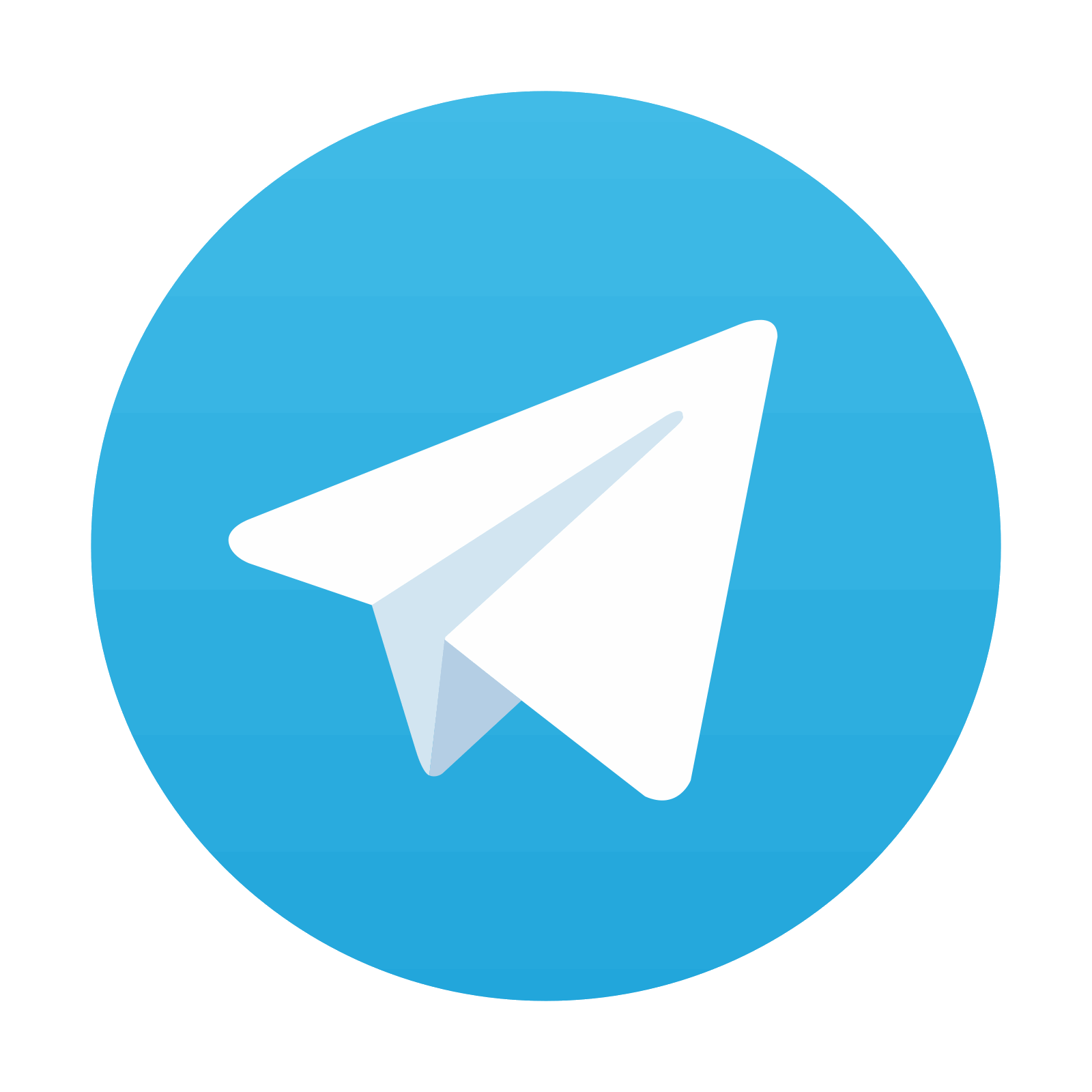
Stay updated, free articles. Join our Telegram channel
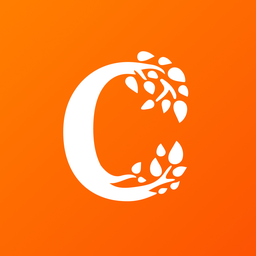
Full access? Get Clinical Tree
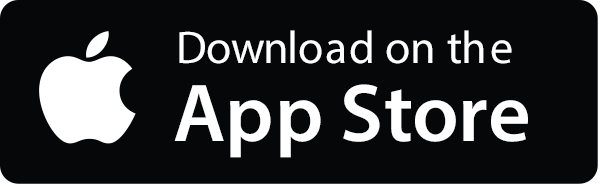
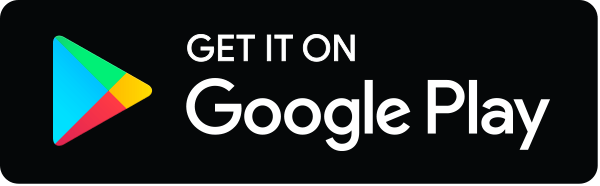