(1)
Department of Clinical Neurological Sciences, Western University, London, ON, Canada
4.1 General Considerations
Electrocortical stimulation of the human neocortex in patients under local anesthesia has now been practiced for over a century, beginning around the turn of the nineteenth to twentieth century, in Britain (Horsley 1886, 1887) and Germany (Krause 1924; Foerster 1926, 1929a, b, 1936a, b; Krause and Schum 1931; Urban 1937). In the middle of the twentieth century, Dr. Wilder Penfield of the Montreal school used the training he had learned from Professor Otfrid Foerster in the use of such stimulation to explore the functional localization of the human cortex in patients who were undergoing surgery for the relief of epilepsy under local anesthesia. His observations and the observations of those whom he trained and who followed him at the Montreal Neurological Hospital and Institute form the most extensive systematic exploration of cortical function in human patients. Their observations still provide the most important contributions to the understanding of the localization of function in the human brain.
Electrocortical stimulatory exploration was established as the gold standard for identifying functional areas within the human cortex and has provided important information to neurosurgeons regarding the safe limits of cortical resection. Outcomes of surgical resections have added to that field, but the progressively increasing sophistication of medical imaging of the brain is now providing a most important array of observational data, which, much more noninvasively, is adding significantly to our understanding of the function of many areas of the brain, especially those areas which have been naively labeled by some as the “non-eloquent” cortex, e.g., that without any obviously apparent clinically important function. For purposes of brevity, the term “eloquent cortex” will be used to indicate the cortex, the stimulation of which produces a clearly identifiable clinical function. However, this use should not be construed to infer that the “non-eloquent” cortex is indeed without any clinical significance.
Electrical stimulation of every area of the human cerebral cortex and in every human being is capable of resulting in the production of an epileptic seizure, even in the hands of experienced individuals who adopt carefully regulated stimulation protocols. Interestingly, the experienced neurosurgeon can often appreciate more early the onset of a seizure compared to less experienced operating room staff that may actually be watching the patient. This may result from some subtle alteration in speech, a motor movement that is below the visual threshold, or some other ill-understood experiential background. Nevertheless, this observation is a very frequent occurrence! It is unimportant as to how a potential seizure is perceived or by whom it is perceived, but it is extremely important to recognize the onset of a seizure as early as possible, in order to ward off a simple partial seizure progressing to a secondary generalization, which can destroy the reasons for operations under local anesthesia, e.g., the presence of a fully alert patient. If the surgeon is aware early on of a beginning seizure and the anesthetist warned about it, then, if necessary, the anesthetist’s preoperatively prepared syringe of the appropriate agent, now most likely propofol, can be injected to abolish it. I have had the experience of such generalization of a seizure occurring when operating in the posterior aspect of the Sylvian fissure before the temporoparietal speech area was appreciably outlined; it took the patient 1 h to wake up, at which point we continued!
Under ideal circumstances the surgeon, in any given operation, can usually gain all the observational data that are required of the stimulation in a matter of tens of minutes. That is to say, 10–20 min of stimulation may be all that is required to obtain the localization of the functional cortex, or the lack of function within the cortex, under observation. In more complex situations this may rise to 30–60 min. During this time, it is important to minimize the risk of creating an epileptic seizure. A very focal seizure may be only a nuisance. However, a generalized seizure, which is allowed to undergo its full course, can result in a postictal altered conscious level that precludes any useful stimulation for an hour or more and indeed occasionally may preclude any further useful stimulation in the operation. This can be catastrophic under some circumstances, including the one I noted in the preceding paragraph.
There are other methods for the “mapping” of cortical function, including the use of evoked potentials, sometimes the satisfactory use of surface stimulation of the motor cortex under general anesthesia, and perhaps eventually the use of the ever improving fMRI imaging. These will not be discussed further.
4.2 Physical and Electrical Characteristics of Stimulation
4.2.1 Stimulus Pulse Waveform
Lilly and his colleagues ushered in a new era of understanding the basis of safe stimulation of the brain in the middle of the last century with the systematic investigation of various aspects of biological stimulation (Lilly et al. 1952, 1955a, b; Lilly 1961). Their primary contribution was a demonstration of the injurious effects of long-term monophasic waveform stimulation of brain tissue. They suggested that the injury was the result of the total charge content of the train of monophasic pulses, as opposed to the peak current of the train. They felt that the very high peak current required for stimulation, when using very brief pulses, could cause thermal injury, while on the other hand, excessively long pulses could result in sufficient transfer of charge over the pulse duration to cause electrolytic injury. They experimented with biphasic waveforms that were “balanced” about zero, thus giving rise to no net transfer of charge (Fig. 4.1). These were shown to cause no tissue damage. This waveform has become known as the “Lilly,” “balanced,” “bidirectional,” or “biphasic” waveform. Further, they demonstrated that the threshold for the production of movement from the motor cortex stimulation was lower with anodal monophasic waveform pulses than with cathodal monophasic pulses, and they further demonstrated that the balanced waveform gave the same thresholds as the anodal pulses. Experimenting with separations (intervals) between the two phases of the waveform of less than 100 μs was attended by higher thresholds, and they finally concluded that ideal stimulation with bidirectional pulses consisted of the two phases of the pulses separated by approximately 100 μs.


Fig. 4.1
The balanced waveform pulse of electrical stimulation. The so-called balanced waveforms, i.e., the charges of the two phases are equal and opposite, result in no net transfer of charge to the tissue being stimulated. This is shown as a rectilinear (square wave) pulse, but the “balance” can be achieved with any waveform, quite irrespective of its shape. (a) The leading phase is the anodal wave; (b) The leading phase is the cathodal wave. (c) The same stimulating pulses of figure (b), but with separation (time) between the two pulses. sep separation (time interval) between the two pulses, x the width (time) of the pulse, {— the current of the pulse
While the balanced waveform is not necessarily important in short periods of acute stimulation, nevertheless, it has led to the preferred use of balanced waveforms in any chronic biological stimulation. MacIntyre et al. (1959, 1960) showed that any lack of balance between the two phases of a bidirectional pulse increased the size of potential lesions at the electrode sites, but demonstrated no lesional effects of separations of the two phases over the range of 0–150 ms (see Fig. 4.1c). They further showed that any waveform, if balanced, gave similar results, e.g., sinusoidal discharges, rectangular discharges, condenser discharges, etc. (see review by Girvin 1978).
4.2.2 Stimulus Parameters
Mihailović and Delgado systematically investigated the parameters of stimulation, applied to the monkey motor cortex, resulting in the threshold of movements, and concluded that biphasic pulses of 0.1–0.2 ms, at 60–150 Hz, were optimal (1956). Lilly and colleagues arrived at similar conclusions with respect to ideal parameters of stimulation, e.g., pulse durations between 0.1 and 1.0 ms and frequencies of about 60 Hz. Libet and colleagues studied the parameters of stimulation in the human somatosensory cortex under local anesthesia and similarly concluded that optimal parameters included train durations of about 1 s, pulse durations of 0.5–1 ms, and frequencies in the range of 15–100 Hz (1959, 1961, 1964, Libet 1973).
The Montreal school used relatively constant parameters of stimulation over the years. These consisted of ~2 ms pulses, at a frequency of 60 Hz, with variable train durations of a few seconds and current strengths produced by outputs of 1–4 V (Penfield and Jasper 1954, pp. 761–2; Rasmussen and Milner 1975). My own preference has been the use of 200–500 μs pulses, at frequencies of 10–50 Hz, train durations of a few seconds, and currents of 1–5 mA. In the stimulation of the cortex, looking for motor responses, I have found that the use of a frequency below that of the fusion frequency of muscle contraction is beneficial, i.e., in frequencies less than 10 Hz. It allows one to observe very fine movements in muscle, which may not be seen at threshold when using higher frequencies and low current; that is to say, it may be disguised by the fusion of subtle contractions.
My own experience has been the same, although much less extensive as the Montreal school in that there has been no specific parameter of stimulation, upon which “the nature of the response is… dependent on any of the parameters tested to date” (Rasmussen and Milner 1975, p. 240).
4.2.3 Electrode Composition
Electrode arrays contain at least two components—the metallic material of the electrodes and the substrate which is carrying the electrodes or in which the electrodes are embedded. In the case of a simple single stimulating electrode, the latter substrate is not terribly important. It has been known for some time that not all electrode metals and substrate components have the same biocompatibility (Dymond et al. 1970). The flow of current from an electrode into biological tissue requires a transformation of the electrical current passing through the stimulating electrode into an ionic (electrolytic) current passing through the excitable tissue. The choice of electrode metal is optimal when the required levels of stimulating current are well below those that cause significant dissolution of the chosen metal.
MacIntyre et al. (1960) demonstrated the striking difference in lesion sizes occurring as a result of the very same stimulation when using copper in comparison to stainless steel electrodes. Those in the former were much more striking. There have been many investigations involving the use of electrodes of varying composition. Many of the observations arising from these studies are anecdotal in that the purpose of the investigations was not directed to a systematic comparison of the metals. Certainly metals such as cobalt, copper, lead, mercury, and silver have all been shown to be significantly toxic to biological tissues. In general, the noble metals have shown much greater biocompatibility. Platinum (Pt) or the much more mechanically strong alloy of platinum and iridium (Pt-Ir) has been accepted as the most biocompatible electrode metal. However, even Pt has been shown to result in tissue damage in experimental animals with excessive stimulation (Agnew et al. 1975, 1977, 1983).
4.3 Variables in Electrical Stimulation of the Human Cortex
4.3.1 Introduction
From most textbook descriptions of stimulation, the reader cannot help but be left with the impression that cortical stimulation and the interpretation of the responses to that stimulation are perfectly straightforward. It is true that in the majority of stimulations, such may be the case. However, there are variations, which can occur and which lend difficulty to interpretations, especially in the initial observations of a novitiate. Some knowledge of these is important in those practices where cortical mapping will be widely utilized. These variations usually can be grouped under certain categories, including (1) stimulus output, (2) unifocal versus bifocal stimulation, (3) responses to threshold versus suprathreshold stimulation, (4) regional differences in threshold, and (5) the potential variability in the reliability and repeatability of cortical responses. All of these require at least mention.
4.3.2 Stimulus Output
It is the flow of current that brings about the stimulation of excitable tissue. Thus, at least intuitively, a constant current stimulator has the best output for such stimulation. However, if the impedance of the stimulating electrode is constant, or relatively constant, then a constant voltage stimulator is just as efficacious in putting out constant current for a given voltage. Over the last century the constant voltage stimulator was much more available than the constant current stimulator, and hence, most of the stimulation studies used the former. This was certainly true until the last quarter of the twentieth century.
4.3.3 Unifocal Versus Bifocal, Stimulating Electrodes
Stimulation can be carried out using a single point of stimulation, referred usually to a large independent electrode remote from the point of stimulation on the cortex, or using two points of stimulation. While these two methods have often been referred to as unipolar and bipolar stimulation, the terms unifocal and bifocal are really more accurate in describing the stimulation, as there are always two poles to the stimulus and thus theoretically all stimuli are bipolar! Unifocal stimulation implies a current density, which is maximal at a single point, i.e., the point of application of a single stimulating electrode. It is characterized by stimulation through an electrode on the cortex with the current path being completed by an indifferent electrode placed elsewhere, e.g., cranium, large areas of exposed tissue in the craniotomy site, areas of the body, etc. In this instance the stimulating current is delivered through the cortex at this single point alone (Fig. 4.2a). The amount of stimulated cortex will be a function of a number of variables, including the strength of the stimulus, the impedance and size of the electrode, and very occasionally to some extent the location of the remotely placed indifferent electrode.


Fig. 4.2
Current pathways in electrocortical stimulation. An illustration of the current pathways associated with stimulation of the cortex. (a) Unifocal stimulation, in which the current is delivered through a single electrode, with the return pathway being that of a significantly larger, remotely placed electrode. (b) Bifocal stimulation, in which the current is delivered through one of a pair of small electrodes, at any given time, with the return path being that of the other electrode. + anode, ─ cathode
In the case of bifocal stimulation, there are two electrodes on the cortex, usually close to one another, e.g., units of millimeters, and usually they are equal in size, construction (metal and substrate), and connecting wires. Thus, the current flow through the two electrodes is equal, and other than being in opposite directions, e.g., anodal and cathodal, the electrodes have the same charge characteristics and current densities. The current pathways are illustrated in Fig. 4.2b.
What is the difference between the two varieties of stimulating electrodes? Either of the two different electrodes can be used effectively. Generally the preference comes down to that with which the surgeon is most familiar, or with which he/she was trained. The bifocal electrode has two points of stimulation, e.g., both the cathode and anode. As outlined in the foregoing text, anodal stimulation usually has a lower threshold than cathodal for the elicitation of responses from the cerebral cortex. While one may not be certain as to which of the electrodes might be responsible for the response, in most cases this is not important, especially when the two electrode points are very close together, as the area stimulated is in the area under and between the two electrode contacts on the surface of the cortex (Fig. 4.2b). Because of the fact that the actual area of the cortex stimulated can perhaps be more easily ascertained, there has been a prevailing view that the bifocal stimulation is more localized, i.e., more discrete. I am not convinced of this.
I have always preferred the use of the unifocal electrode for a number of reasons. (Yes, it is the electrode upon which I was brought up and with which I am the most familiar!) Having used both types of electrodes, I do not feel that the discreteness of stimulation is an issue. It is interesting that the Montreal school initially used “a bipolar electrode made of two small platinum ball tips placed 2–3 mm. apart,” but “more recently we have found it preferable to employ a monopolar electrode with an indifferent contact strapped to the leg” (Penfield and Jasper 1954, p. 761). I believe that there are a number of advantages of the unifocal electrode. It provides less variation in responses to a given stimulation. I have found it easier to place a single electrode in contact with the cortical surface than in placing two electrodes on the cortex such that the pressures and the areas of the electrode contacts are always the same in the two electrodes from one site of stimulation to another. In the absence of the latter, there can be alterations in the impedance and the amplitude of the stimulating current, especially in the case of constant voltage stimulators, which can alter the results of the stimulation. This latter can be altered to some extent by the use of a more mechanically flexible pair of electrodes, but usually this results in a potential difference in the interelectrode distance with varying degrees of pressure of application and thereby again with some variation in the characteristics of the stimuli. Finally, perhaps of most importance is that the single electrode is much more easily placed satisfactorily on an area of the cortex.
4.3.4 Threshold Stimulation
The basic studies involving stimulation of both experimental animals and human patients utilize threshold (T) or “just threshold” stimulation. Using the least amount of stimulating current to cause an effect, i.e., threshold current, is the safest current, for many reasons which will be discussed. Within that context, once a threshold has been determined, there is enough variability in response that each “threshold” (T) stimulus may fail to duplicate exactly the same response from one stimulation to the next. For this reason it is not uncommon to use some stimulus that is just above T, e.g., 1.25 T (25 % above threshold), which generally provides for more stable responses.
It was shown very early on in the cortex of dogs that repeated subliminal, i.e., subthreshold, stimuli, if placed close enough together, may result in a threshold response (Bubnoff and Heidenhain 1881). This phenomenon has become known as summation. From electrocortical stimulation studies in subhuman primates, Sherrington and his colleagues recognized what was referred to as “functional instability of cortical motor points” (Graham Brown and Sherrington 1912; Leyton and Sherrington 1917). They disclosed that as a result of preceding stimulation applied in the same vicinity of the cortex, there could be different responses, which they divided into three categories, (1) facilitation of response, (2) reverse of response, and (3) deviation of response. That is to say, the influence of prior stimulation was able to give rise to a variety of alterations in the normal response to stimulation of the succeeding response; variations did not occur if sufficient time was allowed between the successive stimulations. Penfield and Rasmussen (1968) referred to this variability as “instability of a motor point,” noting the work of Sherrington and his colleagues. Facilitation has been used to describe the summation noted above, i.e., the phenomenon of two successive subliminal responses reaching a threshold response (Grünbaum and Sherrington 1901, 1903). It has also been shown that similar repeated stimulation, at rather longer intervals, may lead to a reduction in response, a phenomenon referred to as extinction (McCulloch and Dusser de Barenne 1935).
4.3.4.1 Regional Differences in Threshold
There are regional differences in threshold estimations. Some of such differences are predictable, while others are less so. The thresholds for the elicitation of motor responses from the motor cortex or somatic sensory responses from the sensory cortex are generally accepted as the lowest cortical thresholds. This has given rise to the common practice of initially determining the threshold of the Rolandic cortex, if it is exposed in the craniotomy, in order to obtain what might likely be a safe level of stimulation in which to initiate an exploratory stimulation. It is particularly important to get some idea of a reasonable threshold stimulation when the exploratory stimulation is to involve the cortex which may not result in clinically recognizable responses, e.g., thus giving rise to the possibility of the use of excessive current strengths resulting in seizures.
Perhaps one of the best examples of regional, or areal, differences in thresholds is that pertaining to the anterior and posterior speech areas. The threshold for the stimulation-induced interference of speech from Broca’s and Wernicke’s areas is higher than that of the Rolandic cortex. The difference may be as high as five- to tenfold. Usually the threshold for such dysfunction in Broca’s area is lower than that in Wernicke’s, but, on the other hand, in any given patient the thresholds may vary widely and in fact the threshold in the cortex of Wernicke’s speech area may even be lower than that in Broca’s area in the odd case.
In some experimentation of penicillin convulsions in monkey cortex, Walker and colleagues concluded that the “reactivity to Penicillin follows closely the electrical excitability of the cortex” (1945), simply reflecting different properties in different areas of the cortex. Similar observations have been made by French and colleagues (1956).
4.3.4.2 Why Are There Regional Differences in Thresholds?
There are many neurophysiological explanations for differences in thresholds from one area of the cortex to another. These are intrinsic properties, which are not pertinent to this discussion. What are pertinent, however, are considerations related to the stimulating equipment, per se, on the one hand and, on the other hand, anatomical variations in the localization of the functional cortex being stimulated. With respect to the former, it is most important that the hardware being used is maintained in satisfactory condition, as this is not something that should ever have to be sorted out in the operating room! However, it is worth noting that commonly the dials on the stimulator are not sterile, in which case it is common for some other member of the operating room staff to be responsible for altering the parameters of stimulation and the strength of stimulation. If this member does not have a reasonable familiarity with the apparatus, the dials associated with the stimulation parameters (pulse waveform, pulse duration, frequency, and in some, train duration), and particularly the current strength, then there can be variations in stimulation and areal thresholds that are not interpreted accurately because of errors in the appropriate settings of the output stimulation—the resulting interpretations therefore being “apparent,” not real.
What can lead to erroneous interpretations in thresholds is the lack of uniformity of the electrical characteristics of the actual cortical surfaces being stimulated. It is important to keep the cortical surface as uniformly moist (saline) as possible, thus keeping to a minimum the possibilities that threshold differences are reflected simply in differences in the impedances of the stimulating electrode, which may be significant when moisture is not present, or contrariwise if there is too much irrigating fluid present. This is particularly important when using a constant voltage stimulator.
Perhaps the most important true alterations in apparent thresholds are due to anatomical variations in the locale of the eloquent cortex. This will be addressed in Sect. 4.5.
4.3.5 The Afterdischarge of Electrocortical Stimulation
Ever since the introduction of electrical stimulation of the mammalian cortex, it has been recognized that if the strength of such stimulation is raised sufficiently, seizures can nearly always be produced. The ECoG (electrocorticogram) in the region of the stimulation (or the ECoG if the stimulation is that from an earlier placed subdural array of electrodes) allows the observation of the cortical electrical activity and any alteration of the activity resulting from the stimulation. Under this circumstance, “after-discharges”, which consist of post-stimulus, e.g., beyond the cessation of stimulation, abnormal electrical activity in the area of stimulation may be observed. Afterdischarge very rarely might occur with near-threshold stimulation, but with increasing strengths of stimulation, the probability of occurrence of afterdischarges rises. Some type of afterdischarge nearly always precedes seizures produced by electrocortical stimulation. This afterdischarge may assume a variety of morphologies, e.g., rhythmic waves, polyspike bursts, sequential waves etc., which may be seen with one another, may change from one to another, and may be observed in any part of the area surrounding the point of stimulation (see Blume et al. 2004).
As indicated in the foregoing, under the usual circumstances of subthreshold or just at threshold stimulation, there is nearly never stimulation-induced electrical activity outlasting the actual period of stimulation. This is demonstrated in Fig. 4.3a. The recording of the cortical activity is lost during the artifact of the stimulation, per se, as well as its following brief blockage of the recording apparatus, but following this the recording is re-established. When the strength of stimulation is elevated above threshold, especially when excessive, the appearance of abnormal artifactual electrical activity may be seen in the stimulated cortex and outlasting the period of stimulation. Since rhythmic activity is the commonest abnormality seen in the afterdischarge, this will be illustrated in Fig. 4.3b, c. Afterdischarge, occurring in eloquent cortex, may be associated with a clinical abnormality, e.g., the interference, positively or negatively, with the clinical function of the area. While this afterdischarge is self-sustained, it is usually transient with small increases in stimulation current (see Fig. 4.3b). That is to say, the afterdischarge is self-limited and thus whatever clinical alteration might have occurred will similarly be self-limited as well. In this circumstance the electrocortical abnormality will be localized, primarily to the area of the stimulated cortex. As higher stimulus strengths are applied, the afterdischarge may become fully self-sustained, as shown in Fig. 4.3c. Under this circumstance the electrical activity may spread to the adjacent cortex, may be associated with more vigorous electrographic epileptic activity (see Erikson 1940), and may exhibit evidence of clinical epileptic activity that is likely related to the functional cortical area being stimulated. As indicated earlier, this very same sequence may be seen from stimulation of the non-eloquent cortex, the clinical epileptic activity either starting locally, as a result of the spread of the afterdischarge to a nearby area of the eloquent cortex, or even in the form of a typical generalized grand mal seizure. If the seizure is not stopped by the administration of anesthetic or pharmacologic means, when it is clearly progressing, then it will usually end up in a generalized full-blown convulsion.


Fig. 4.3
Afterdischarge following electrocortical stimulation. (a–c) each discloses the initial normal electrical activity (ECoG) of a region of the cerebral cortex and the alterations that occur in the ECoG as a result of increasing strengths of applied electrical stimulation. Each of the figures demonstrates the initial normal ECoG, the marked abnormal artifact from the 0.5 s electrocortical stimulation, followed by a ~1–2 s of no electrical activity as a result of the transient blockage of the ECoG by the stimulation. Following these three fixed alterations are different responses, as a result of increasing strength of the current of the stimulation. (a) depicts the result of a stimulus at threshold, which does not alter the normal activity, i.e., it is not associated with any abnormal discharge after the termination of the effect (artifact and ECoG blockage) of the cortical stimulation. (b) illustrates the result of an increase in the current of the stimulation, such that it leads to a transient self-sustained, self-limited, afterdischarge. (c) illustrates a further increase in the stimulation current that is strong enough to produce a self-sustained afterdischarge, which progresses and spreads to the nearby cortex and often eventually results in an epileptic seizure. μV microvolts, st.art. stimulation artifact
Walker (1949a, b) found that the lowest thresholds for the production of afterdischarges were associated with those areas of the cortex in and about epileptic foci in patients with intractable seizures. He referred to this cortex as “hyperirritable” or “hyperexcitable.” Putting aside the foregoing it must be remembered that with sufficient electrical stimulation, nearly any area of the cortex can be made to give rise to afterdischarges and epileptic seizures.
Just as there are regional variations in threshold responses in the eloquent cortex to electrocortical stimulation, there are variations in the thresholds for the production of afterdischarges. For example, the threshold for the production of the latter is much lower for the Rolandic cortex than for the non-eloquent cortex such as that in the anterior temporal and frontopolar regions. The characteristics of the afterdischarge, e.g., asynchronism, synchronism, rhythmicity, types and frequency of waveforms, etc., may vary widely from one area of the cortex to another.
To summarize, once afterdischarge occurs, it may (1) be a self-limited discharge, disappearing in a matter of a few to tens of seconds; (2) remain very localized, transient, and stationary for a significant period and even in this state can demonstrate clear focal epileptic discharge and associated clinical focal seizure activity; (3) spread and again terminate in a self-limited fashion; or (4) spread, in association with spreading clinical epileptic activity, which terminates in a full-blown generalized seizure. Penfield and Jasper (1954) have also shown that remote afterdischarge can occur, e.g., afterdischarge that may be some centimeters away from the stimulated area. This is not seen often and is not really very well understood. As outlined in the foregoing section on threshold stimulation (Sect. 4.3.4), subthreshold stimulation, rapidly repeated, can lead to summation, evidenced by the appearance of a threshold response, even though the stimulus, which finally elicited it, was subthreshold. This same phenomenon of summation may occur with afterdischarges. Thus, a series of subthreshold stimuli, repeated sufficiently close together, can result not only in a threshold response but even in the summation of afterdischarges, which, like any afterdischarge, may reach sufficient strength to be associated with an epileptic seizure.
I would be remiss in not indicating that in practice the majority of careful stimulations are not associated with any afterdischarges and that when they do occur they are self-limited and without an accompanying ECoG abnormal activity; the surgeon would be unaware of any abnormality. However, during sessions of electrocortical stimulation, the surgeon must continually bear in mind the possibility that afterdischarge may be continually produced and thus be prepared, along with the anesthetist in charge, to take whatever precautions that are necessary to avoid the production of seizures, especially generalized seizures (vide infra), which can interfere with the gathering of the information, which was the primary objective of the use of local anesthesia.
4.3.6 Isolation of Current Input
Isolation of the stimulus input to human tissue is required in most jurisdictions. This has become more important down through the years to the point that now some stimulators have the isolation available within the stimulator per se. Isolation may be carried out in a number of ways, e.g., transformer, capacitor, optical, etc. There is no necessity to describe the various strategies in detail. The importance of this can be found in the literature (Dobelle and Mladejovsky 1974; Dobelle et al. 1974).
4.4 The Conduct of Exploratory Stimulation
4.4.1 Introduction
Exploratory stimulation really applies to the initiation of the objective of mapping the cortex in question in order to determine the localization of function within that cortex. It will be the knowledge of where this function is located, which will allow the surgeon to determine the safe limits of resection in and about its location. An associated objective of the exploration is the mandatory precaution of minimizing the likelihood of the production of seizures from the stimulation.
It is important at the outset of the exploration to explain to the patient that the stimulation of the cerebral cortex is not painful. Many patients who hear that they are to undergo a stimulation consisting of “electrical” stimuli immediately interpret the stimulation as being associated with a number of electric shocks. A more relaxed patient will be achieved by making certain that such an interpretation is precluded.
Communicating with the patient such that she/he knows when the stimulus is being applied is perhaps the most important methodological issue to be addressed in routine mapping of the functional cortex. The patient must know exactly when the stimulus is being applied. Failure to satisfy this feature of stimulation leaves responses which are usually associated with tremendous uncertainty. I have usually used the word “now” in some context. Commonly, I will say something to the effect that “the stimulus is coming …. now,” but nearly always end up simply saying “coming …. Now,” applying the electrode to the cortex exactly coincident with the “now.”
4.4.2 Minimizing the Likelihood of Stimulus-Induced Seizures
As noted in the foregoing, it is most important to avoid the production of generalized seizures. Thus, it is the prophylaxis, rather than the management, of these seizures that should be the foremost strategy. Prophylactic initiatives include (1) the use of low currents of stimulation, (2) the employment of random spatial stimulation, (3) optimal temporal spacing of successive stimuli, (4) the “no-return-to-a-previously-delineated-area” policy, (5) the use of ECoG monitoring, and (6) anesthesia-on-the-ready.
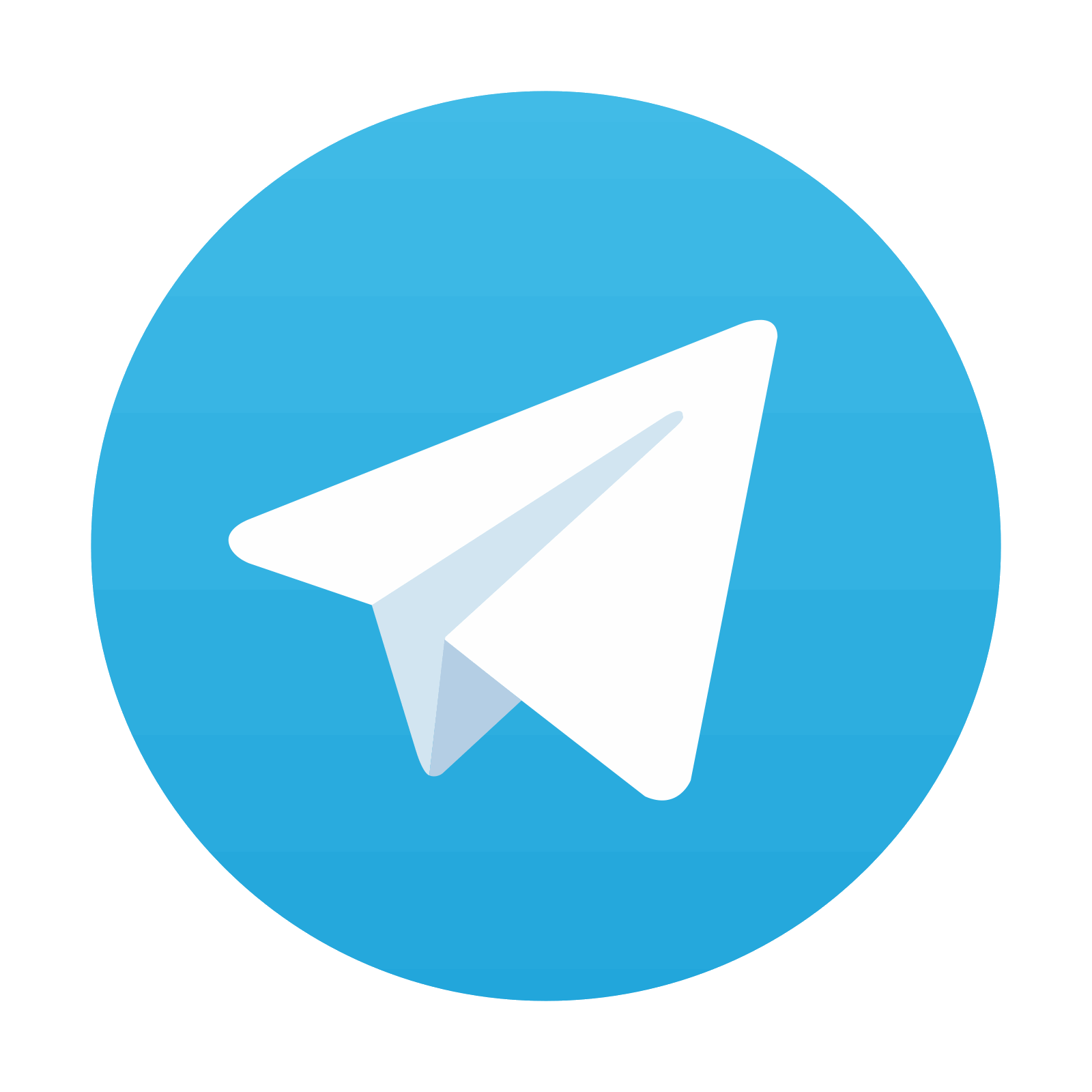
Stay updated, free articles. Join our Telegram channel
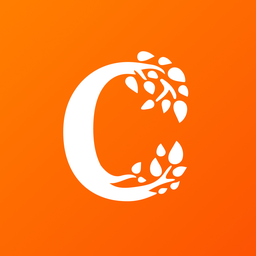
Full access? Get Clinical Tree
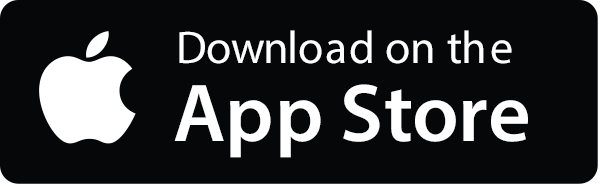
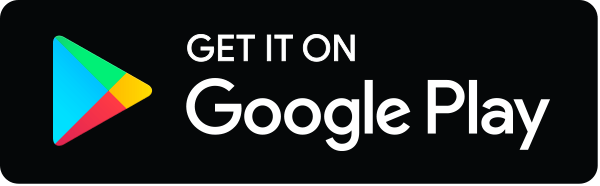