Abstract:
The goal of this chapter is to enhance the clinician’s ability to recognize indications for the most commonly used electrophysiological tests and to integrate knowledge of these test indications and findings into the management of patients with neuropathological or myopathic dysfunction. The first section presents a basic description of the electrophysiological tests, including nerve conduction studies (NCSs), electromyography (EMG), kinesiological electromyography (KEMG), and the underlying neuroanatomical structures being tested. Normal and abnormal findings are discussed, with emphasis on how knowledge of these tests can assist the therapist in patient evaluation. The second section provides an introduction to the physiology, indications, contraindications, equipment, and applications of electrical muscle stimulation (EMS), neuromuscular electrical stimulation (NMES), and electromyographic biofeedback (EMGBF). The information integrates electrotherapeutic interventions into program planning for common neurological body system problems, their subsequent functional limitations, and perceived decrease in quality of life. Published evidence examining efficacy is included to assist the therapist in making choices about the use of these tools in the clinic. The third section provides a series of four case studies that illustrate the usefulness of electromyographic testing in patients with a variety of medical diagnoses.
Keywords:
econtilectromyographic feedback, electroneuromyography, functional electrical stimulation, kinesiological electromyography, nerve conduction velocity, neuromuscular electrical stimulation
Objectives
After reading this chapter the student or therapist will be able to:
- 1.
Identify electrophysiological tests performed on patients with neurological or muscle disorders.
- 2.
Describe the instrumentation and general procedures for electrophysiological testing.
- 3.
Recognize normal and abnormal findings of various electrophysiological tests.
- 4.
Recognize the differences in instrumentation, signal processing, and interpretation when performing electrophysiological testing versus kinesiological electromyographic testing.
- 5.
Differentiate the basic mechanism underlying functional neuromuscular stimulation, electrical stimulation, and electromyographic biofeedback.
- 6.
Describe the appropriate instrumentation, signal processing, and interpretation for kinesiological electromyographic testing.
- 7.
Describe the indications and contraindication for the use of neuromuscular stimulation, electrical stimulation, and electromyographic biofeedback.
The goal of this chapter is to enhance the clinician’s ability to recognize indications for the most commonly used electrophysiological tests and to integrate knowledge of these test indications and findings into the management of patients with neuropathological or myopathic dysfunction. The first section presents a basic description of the electrophysiological tests, including nerve conduction studies (NCSs), electromyography (EMG), kinesiological electromyography (KEMG), and the underlying neuroanatomical structures being tested. Normal and abnormal findings are discussed, with emphasis on how knowledge of these tests can assist the therapist in patient evaluation. The second section provides an introduction to the physiology, indications, contraindications, equipment, and applications of electrical muscle stimulation (EMS), neuromuscular electrical stimulation (NMES), and electromyographic biofeedback (EMGBF). The information integrates electrotherapeutic interventions into program planning for common neurological body system problems, their subsequent functional limitations, and perceived decrease in quality of life. Published evidence examining efficacy is included to assist the therapist in making choices about the use of these tools in the clinic. The third section provides a series of four case studies that illustrate the usefulness of electromyographic testing in patients with a variety of medical diagnoses.
Electrophysiological testing
Physical therapists (PTs) are uniquely positioned to understand and perform electrophysiological testing. This is because PTs examine patients with pain, numbness, and/or weakness and also because they have a superior knowledge of neural and muscular anatomy and physiology. Electrophysiological testing is a sensitive and specific tool designed to assist in the diagnosis and the development of treatment plans for patients with diseases of the peripheral nervous system (PNS) and of the muscle itself.
An informed perspective on the application of these tests will benefit the physical therapist’s interaction and communication with other members of the medical team. At the completion of the electrophysiological consultation, the clinician who performed the test generates a report. Understanding this report can guide decisions in planning and modification of intervention programs or will assist in referring to other health care practitioners. A review of components of such report follows.
Electrophysiological tests are usually performed by neurologists, physiatrists, and PTs who have education, training, and experience in these procedures. Most PTs practicing in the area of clinical electrophysiology are board certified by the American Board of Physical Therapy Specialties. Some states (such as California) require additional licensing.
The general goal of electrophysiological testing is to answer the following questions:
- •
Is there a lesion in the PNS and/or muscles?
- •
Where precisely is that lesion (nerve, neuromuscular junction, muscle)?
- •
What is the extent of the lesion (myelin, axons or both)?
Most of the electrophysiological tests described involve the application of an external electrical stimulus to a nerve or muscle and observation and assessment of the muscle or nerve response. Other tests such as needle EMG and single-fiber electromyography (SFEMG) involve the monitoring and recording of the electrical activity produced by the muscle tissue at rest or during contraction.
The electrophysiological tests most commonly used are motor and sensory NCSs, including F-wave and H-reflex latency measurements; repetitive stimulation; somatosensory evoked potential (SSEP) tests; and needle EMG. Most often, a patient referred for electrophysiological testing will undergo at least two motor nerve conduction tests, at least two sensory conduction tests, and at least one limb needle EMG. The American Association of Neuromuscular and Electrodiagnostic Medicine (AANEM) has published evidence-based guidelines, which may be found on the AANEM website at http://www.aanem.org/Practice/Practice-Guidelines . A review of the patient’s history, a relevant systems review, and a physical examination guide the examiner in the selection and sequencing of appropriate tests. In other words, muscle strength and tone, sensation, range of motion (ROM), cranial nerve assessment, reflex testing, neurological signs, and cognition are crucial in selecting and administering electrophysiological tests. Most subject matter experts consider electrophysiological testing as an extension of the clinical examination. , It does not replace a careful history and physical examination of the patient. It does, however, establish the precise state of the nerves and muscles and can thus determine the location of a lesion more precisely than the clinical examination alone, particularly in cases of mild weakness or ill-defined sensory changes. In very clearly defined pathologies, electrophysiological tests are not necessary (except perhaps for medico-legal reasons). For example, in the case of a unilateral ankle dorsiflexion weakness coupled with a clearly defined L5 nerve root compression on magnetic resonance imaging (MRI) of the lumbar spine, the electrophysiological test may be of little added value. However, for a similar clinical presentation (foot drop) and no clear-cut imaging, the electrophysiological tests will differentiate between a fibular palsy, a sciatic nerve neuropathy, a lumbosacral plexopathy, or an L5 radiculopathy.
Finally, evidence-based practice recommends that the practitioner have a good understanding of the implication of the sensitivity and specificity of each test to rule it in or out for a specific condition.
Anatomical review
In order to best understand the systematic interpretation of data from the electrophysiological examination of nerves, the reader is invited to review the following foundational principles. These are explored in much greater detail elsewhere in this text.
At the cellular level
A nerve is composed of axons covered with a sheath of myelin. Depolarization inside the axon is an “all-or-nothing” phenomenon in which an action potential moves along the surface of the cell membrane. This action potential is an electrical wave caused by a flow of ions across the cell membrane. A local current opens a sodium channel, allowing Na + ions to rush inside the cell. The electrical resistance to this wave is inversely proportional to the diameter of the axon. Larger nerves conduct faster than smaller nerves. In order for efficiency as an organism to be achieved, nerve conduction must be fast. In complex organisms with billions of axons, increasing the nerve diameter is not a viable option; hence, the role of the myelin sheath. The myelin is produced by Schwann cells. These are special satellite cells that separate axons from the endoneural fluid. The myelin acts as a capacitor: the conduction “jumps” between gaps in the myelin called nodes of Ranvier. This saltatory conduction allows human nerves to be 50 times smaller but conduct four times faster than unmyelinated nerves. Consequently, recording and analyzing the conduction velocity of nerves primarily reflect on the state of the myelin. The amplitude of the response (if a supramaximal stimulation is delivered) is a reflection of the number of axons available to the stimulation.
The physiology of the nerve is such that when there is an injury to the axon, the portion of the axon distal to the injury will degenerate (Wallerian degeneration). This is important because all muscles innervated by branches of the nerve distal to the lesion will show signs of denervation approximately 11 days after the lesion. Consequently, assessing a patient too early after a lesion may lead to false-negative results. ,
At the anatomical level
The accurate performance and interpretation of the electrophysiological test—particularly the needle EMG—is significantly contingent on knowledge of the precise innervation of each muscle. As an example, an ulnar neuropathy at the elbow (UNE) clinically may be indistinguishable from a C8 radiculopathy. However, the astute clinician will remember that the cell body of the sensory nerve lies in the dorsal root ganglion, which is typically not involved in a radiculopathy. The therapist will also know that the abductor pollicis brevis is a C8- and median nerve–innervated muscle. Consequently, an ulnar nerve neuropathy is distinguishable from a C8 radiculopathy in that the ulnar sensory test will have decreased amplitude in the UNE and the abductor pollicis brevis will be denervated in the C8 radiculopathy. As a matter of fact, the clinician will keep in mind the innervation of each muscle while conducting the test. Electrophysiological testing is hence a dynamic process during which the choice of the next nerve to test or muscle to sample is predicated on the result of the previous test. ,
As a result, the electrophysiological examination is not a single, stereotyped investigation but an evolutionary one during which several tests (nerve conduction, both sensory and motor, and EMG of several muscles) can be applied to a clinical presentation. ,
Nerve conduction tests
A general overview of NCSs is presented to provide an understanding of their application and indications. Many excellent texts are available for details of the techniques.
Motor and sensory NCSs can provide data that are helpful in establishing the presence and location of pathological conditions in the PNS. The tests may indicate the anatomical level, such as a plexopathy, versus a localized peripheral mononeuropathy. Individual and multiple nerves may be assessed, and the findings compared with responses of the same nerves contralaterally. The site of pathology may be localized, such as median nerve compression at the wrist versus a lesion of the lateral cord of the brachial plexus.
Nerve conduction velocity is faster in myelinated fibers because of saltatory conduction. Disorders involving peripheral demyelination can thus be differentiated from impairments primarily involving axonal degeneration. A mild localized compressive disorder (neurapraxia) may be distinguished from a more severe lesion in which the axons and surrounding connective tissue have been completely disrupted (neurotmesis). , In the event that the findings of nerve conduction studies (NCS) and EMG are normal, the clinician may be able to rule out most conditions involving the PNS and look for central nervous system (CNS) or other pathology. Knowledge of the rationale for NCSs and EMG should help the therapist decide when the tests may be indicated and understand the reasoning behind reports of tests that have already been performed on patients.
Motor nerve conduction
In motor NCSs the peripheral nerve is stimulated at various sites and the evoked electrical response is recorded from a distal muscle supplied by the nerve (a measure of orthodromic conduction). Surface electrodes are usually used for both stimulating and recording. An example of electrode configuration for a motor NCS is shown in Fig. 31.1 (ulnar nerve study). The response represents the electrical activity of muscle fibers under the recording electrodes and is called the compound muscle action potential (CMAP). It is also called the M wave or M response. Measurements are taken of the latency (the time in milliseconds required for the impulse to travel from each stimulus site to the recording site) and the amplitude of the response in millivolts (mV). The shape and duration of the response are assessed, and motor nerve conduction velocity is calculated for each segment of interest by dividing the distance between stimulus sites (in millimeters) by the difference in latency measured at each respective site.

Velocities, latencies, and the shape and amplitude of the responses ( Fig. 31.2 ) are studied and compared with established normal values and often with values taken from tests of the uninvolved extremity (when possible). In infants and children, nerve conduction is slower than in adults and reaches adult values by age 4 years. Nerve conduction velocities gradually slow after 60 years of age but generally remain within the outer limits of normal. ,

Sensory nerve conduction
Sensory nerve conduction can be measured from many superficial sensory nerves, such as the superficial radial and sural nerves. It can also be measured from mixed motor and sensory nerves. The stimulus is applied over the nerve in question, and the recordings taken from electrodes placed over a distal sensory branch of the nerve. The recordings are called sensory nerve action potentials (SNAPs). An example of recording and stimulation sites is shown in Fig. 31.3 . Both orthodromic and antidromic conduction can be assessed. Response latencies and amplitudes are measured, and sensory nerve conduction velocities are calculated for each segment by dividing the distance between two adjacent stimulus and recording sites, or two stimulus sites, by the latency (conduction time) between these same sites. Sensory nerve responses are considerably smaller than motor responses. Their amplitudes are generally measured in microvolts (μV). Sensory conduction velocities may be calculated with just one stimulation as, contrary to motor studies, there is no neuromuscular junction to account for in sensory nerves. Sensory recordings are more sensitive than motor recordings in cases of mixed sensory-motor neuropathies.

F-wave latency
When a motor nerve is stimulated in the periphery, both orthodromic (peripherally to the muscle) and antidromic (centrally toward the spinal cord) impulses are generated. A proportion of the antidromic impulses will, as it were, “bounce off” the axon hillock and return as a recurrent discharge along the same neurons to activate the muscle from which the recording is taken. This activity is termed the F wave ( Fig. 31.4 ), and it is observed as a small wave occurring after the M wave. , , No synapse is involved. Thus the F wave is not a reflex response, but rather only a measure of conduction along the motor neuron. Specific conditions of electropotential must exist at the soma-dendritic cell membrane to reactivate the efferent axon; therefore the occurrence of the F-wave response is inconsistent and variable in latency and waveform.

The F-wave latency can be useful in evaluating conduction in conditions usually involving the proximal portions of the peripheral neurons (e.g., radiculopathy, Guillain-Barré syndrome, or thoracic outlet syndrome [refer to Chapter 18 ]). Its value, however, has been questioned by some authors because of its variability. Normal values of F-wave latency are 22 to 34 msec in the upper extremity (stimulating at the wrist) and 40 to 58 msec in the lower extremity (stimulating at the ankle), depending on the height of the subject, with a bilateral difference in latency of no greater than 1 msec.
H-reflex response
The H-reflex response latency ( Fig. 31.5 ) is a measure of the time for action potentials elicited by stimulating a nerve in the periphery to be propagated centrally over the Ia afferent (sensory) neurons to the posterior horn of the spinal cord, to be transmitted across the synapse to α in the anterior horn of the spinal cord, and then to travel distally over these neurons to activate the muscle. The response therefore measures conduction in both the afferent and efferent neurons. , It is also referred to as a “late” response (the other being the F wave).

The H reflex is constant in latency and waveform, and it occurs with a stimulus usually below the threshold level required to elicit the M-wave response (Ia afferent fibers are larger in diameter than α and thus more sensitive to electrical stimulation [ES]). This monosynaptic reflex response is most easily found by stimulating the tibial nerve at the popliteal area and recording from the soleus muscle. Braddom and Johnson reported a mean latency of 29.8 msec (±2.74 msec) for the tibial nerve in normal adults, and a bilateral difference of no more than 1.2 msec. The H-reflex latency is a valuable measure of conduction over the S1 nerve root in differentiating suspected proximal plexopathy and radiculopathy from a herniated disc or foraminal impingement. Sabbahi and Khalil have reported a technique for recording the H reflex from the flexor carpi radialis muscle when stimulating the median nerve. In normal human beings older than 1 year, the H reflex is usually seen only in the tibial, femoral, and median nerves. It can be elicited from several nerves in infants and in conditions of CNS dysfunction in adults.
Repetitive stimulation tests
The repetitive nerve stimulation (RNS) test is used to evaluate transmission at the neuromuscular junction (motor synapse) in patients with diffuse weakness. RNS tests are helpful in the differential diagnosis of disorders such as myasthenia gravis and Lambert-Eaton myasthenic syndrome (LEMS). One protocol uses a series of supramaximal electrical stimuli applied to a peripheral nerve at a distal site (e.g., median or ulnar nerve at the wrist) at a rate of three to five per second for five to seven responses. Changes in amplitude of the muscle response are assessed. Precise technical requirements are specified to prevent movement artifacts and other testing errors. Detailed descriptions of the RNS test can be found in other texts. , Under normal conditions the amplitude does not change more than 10% from that of the initial response in a series of 10 stimuli recorded before and after resistive exercise. An amplitude decrease in the fifth or sixth response of more than 10% is considered abnormal and is compatible with a physiological defect at the postsynaptic receptor site of the neuromuscular junction, as in myasthenia gravis. Because myasthenia gravis most often involves facial muscles, a muscle of choice of the RNS is the nasals muscle.
In another RNS protocol, stimuli are applied to a nerve, first at a slow rate, then at a faster rate, usually 10 to 20 per second for up to 10 seconds. Normally, the amplitude can decrease up to 40% from the initial amplitude. In some defects at the presynaptic site, the response may be lower than normal during a slow stimulation rate but show a significant amplitude increase at the higher rate. Increases in amplitude greater than 100% over the initial response are consistent with presynaptic neuromuscular junction defects such as seen in LEMS, which has a strong association with small-cell bronchogenic carcinoma, and in botulism. In 1957 Eaton and Lambert reported this phenomenon as a myasthenic syndrome.
Gilchrist and Sanders reported another protocol referred to as a double-step RNS test. This test measures amplitude before and after a temporarily induced ischemia of the extremity. They found the double-step RNS test to be slightly more sensitive than the routine RNS test, but only 60% as sensitive as the SFEMG technique. The RNS test is a good alternative test for neuromuscular transmission when the SFEMG is not available, but the examiner must meticulously adhere to technical details when conducting the test.
Blink reflex
In some conditions (e.g., facial palsy) or to establish the presence of a widespread neuropathy, it may be necessary to assess the conduction in the cranial nerves. The stimulation of the supraorbital nerve (branch of the trigeminal nerve, cranial nerve V) elicits two separate responses of the orbicularis oculi muscles, one early and ipsilateral (R1) and one late and bilateral (R2), via the facial nerve (cranial nerve VII). The early R1 response involves a synapse in the pons, whereas the late bilateral R2 responses involve a more complex pathway involving the pons and lateral medulla. Analysis of the respective latencies of each response will help in determination of the following:
- •
Trigeminal neuralgia (cranial nerve V) or Guillain-Barré: all responses delayed
- •
Acoustic neuroma or Bell palsy (cranial nerve VII): ipsilateral responses delayed
- •
Pontine lesion: absence of R1
- •
Medullar lesion: absence of R2
Hence, the blink reflex is a nerve conduction test that assesses a portion of the CNS and has shown some utility in assisting in the diagnosis of multiple sclerosis (see Chapter 19 ) and Wallenberg syndrome.
Clinical evoked potentials
Electrical potentials elicited by stimulation of nerves or sense organs in the periphery can be recorded from various sites as the impulses are transmitted centrally along the neuronal pathway and from the representative area of the brain. , SSEP procedures are particularly useful in assessing the integrity of afferent pathways in the CNS. They are helpful in differentiating among lesions in areas such as the plexus, spinal cord, brain stem, thalamus, and cerebral cortex. Evoked potential tests have the advantage of providing data about the integrity of both peripheral and central neuronal pathways, including transmission across axodendritic synapses.
The SSEP is valuable in assessing damage and continuity of spinal cord tracts in early spinal cord injury (SCI). For example, if an electrical stimulus is applied at the popliteal area over the tibial nerve, responses can be recorded with surface electrodes placed over the spine at the L3 and C7 spinal segments and from the lumbar representation of the contralateral sensory cortical area. Conduction time and other parameters of the response waveforms can be measured from the recordings.
This simplified example of an SSEP illustrates how conduction over sensory peripheral nerves and afferent pathways to the cerebrum can be studied. The median nerve is usually tested to evaluate the integrity of peripheral and central pathways and their synaptic connections as the impulses travel from the upper extremity to the contralateral cortical area.
In visual evoked potential (VEP) procedures, visual stimuli such as variable light flashes of changing patterns are applied to one or both eyes under highly controlled conditions. The response is recorded from the scalp over the representative area of the cerebral cortex. , , The term pattern reversal evoked potentials (PREPs), a more descriptive term for these procedures, is recommended by the American Electroencephalographic Society. These tests and other VEP procedures are useful in assessing pathology of retinal photoreceptors, the optic nerve, and postchiasmal pathways. Abnormal conduction findings have been reported when VEP studies are used in demyelinating disorders such as multiple sclerosis and optic neuritis. The examiner may conclude that the patient is cortically blind because no response is recorded on the visual cortex. Although many causes for a stimulus not reaching the visual cortex are possible, the end result is considered blindness. If the cause for cortical inactivity is swelling or a neurochemical imbalance within a nuclear relay structure, once corrected, the individual may experience normal vision. A change in the reaction of the patient to the visual environment may reflect increased awareness and a change in coma scale rating. Similarly, just because an individual turns toward a light or visual stimulus does not mean an evoked potential reaches the visual cortex. Instead, the eyes as receptors and the visual tract to the brain stem may be intact even though a problem in the synaptic connections between or within the thalamus and visual cortex may exist.
Auditory evoked potential tests are used to evaluate neurological function of the cochlear division of the auditory nerve (eighth cranial nerve), central auditory pathways and synapses in the brain stem, and the receptor areas on the cerebral cortex. , Brain stem auditory evoked potentials are frequently referred to as BAEPs. A series of high-intensity clicks is applied to auditory receptors in the ears through headphones, and several components of the response waveforms are recorded by using surface electrodes over the representative cortical areas. The BAEP is an effective test procedure for localizing and evaluating acoustic neuromas and other space-occupying lesions in the brain stem. This test is also used for assessment of brain damage in patients who are comatose as a result of traumatic brain injury (TBI). Robinson and Rudge recommend caution in using BAEP tests for this purpose because other factors, such as defective receptor organs, can cause abnormalities in BAEPs.
The evoked potential tests described in this chapter all require application of appropriate external stimuli that are rapidly repeated many times. The response is electronically averaged to sort out the desired signal from interference signals. The conduction times (latencies), waveform shape and amplitude, and sometimes conduction velocities are measured and compared with normal values. Absence of a response, increased latencies, decreased amplitudes, and slowing of conduction velocities are all abnormal findings. Normal values and details of techniques for the evoked potential tests are described elsewhere. ,
Therapists with special interest and training administer the SSEP tests for neurological applications more frequently than other types of evoked potential tests. Because of the highly specialized techniques necessary to administer VEP tests for ophthalmological applications and BAEP tests for hearing dysfunction, they are usually performed by persons who specialize in these procedures.
Needle electromyography
Needle EMG complements the motor and sensory NCSs; it is most sensitive in the detection of denervation.
Unlike NCSs, which use the ES of the motor nerves to elicit muscle contraction, the needle EMG is used to record and analyze muscle activity at rest and during voluntary activation. It is particularly useful in identifying pathology of the lower motor neurons and of the muscle itself. EMG can also be used to identify abnormalities of motor neuron recruitment that are associated with certain disorders of the CNS, especially when NCS findings are normal—as would be the case in radiculopathies. The primary recording studied is the insertional activity, along with activity at rest and the motor unit action potential (MUAP), which is produced by the depolarization of single motor units during voluntary or reflex activity. Spontaneous electrical activity of single muscle fibers at rest is termed fibrillation and is diagnostic of denervation. For recording of muscle activity, small-diameter needles are inserted within the muscles to be studied. Three electrodes are required: active (negative), reference (positive), and ground. The needles may be monopolar, requiring a second needle or surface electrode for reference, or bipolar, containing both the active and reference electrodes (usually concentric in cross section). The ground electrode is typically placed on the surface of the skin. Most commonly the needles used are disposable. The activity detected in the muscle is displayed on the video display terminal of a computer (and can be stored and printed later). It is simultaneously played through an audio amplifier. The electromyographer can often identify pathological conditions by the characteristic “sounds” of the electrical activity of the muscle. Many excellent resources are available for readers interested in details of the equipment and procedures for EMG. , , Details of contraindications and special precautions are described by Currier and Nelson.
In an EMG examination, four conditions are evaluated at each location: (1) activity during needle insertion (normally a brief burst [250 msec at most] of high-frequency activity that abates when the needle stops moving), (2) activity during rest (electrical silence is normal unless an electrode is placed directly over a motor endplate), (3) activity during minimal and gradually increasing voluntary contraction (biphasic or triphasic MUAPs of small amplitude composed of slow-twitch type I fibers that increase in frequency and are joined by higher-amplitude potentials as larger, predominantly fast-twitch type II motor units are recruited), and (4) activity during maximal activation (an interference pattern caused by the blending of potentials in which individual MUAPs cannot be identified), characterized as full (complete), reduced, or absent. Several locations in an individual muscle may be studied. The specific muscles to be studied are determined by clinical findings, and results must always be interpreted in the context of the total complex of signs and symptoms. In determining the specific location of a lesion, muscles located both proximally and distally to the suspected lesion site must be assessed. Studies may be repeated at intervals to determine if changes consistent with recovery (such as reinnervation) or exacerbation are present. EMG is typically used to help determine the presence (and extent) of the following:
- •
Denervation
- •
Reinnervation
- •
Myopathic or neuropathic signs
- •
Distribution or specific location of peripheral nerve pathology
Needle EMG is sensitive in determining the state of the axons. When axons are interrupted, there is denervation of the muscle cell. The findings in denervation and partial denervation include increased insertional activity, fibrillation potentials or positive sharp waves at rest, and a reduced or absent interference pattern. CNS dysfunction can result in no resting potentials, but if motor control was impaired, a decreased or abnormal interference pattern might be apparent because of difficulty in recruitment ( Fig. 31.6 ). Needle EMG also informs the clinician about muscle cell disorders such as myopathies. The primary finding in the case of a myopathy is fibrillation potentials and small-amplitude polyphasic MUAPs.

Although patient cooperation during EMG testing is important, some aspects of muscle electrical activity can be studied in the patient who is very young (infant), who is unable to move, or who has only involuntary or reflex activity. Insertional and resting potentials can always be evaluated. MUAPs appear during the contraction of muscle fibers activated both voluntarily and involuntarily in both isotonic or isometric conditions. In normal conditions MUAPs are seen with voluntary movement; however, reflex activation of muscle also produces MUAPs with certain normal characteristics. In CNS disorders, hypertonic or spastic muscles will produce recognizable MUAPs when they are actively contracting. The electromyographer can elicit a contraction by tapping on the muscle or tendon. For example, consider a patient who is recovering from a traumatic head injury with residual spastic hemiplegia. She is unable to cooperate with the EMG exam. The patient has abnormal extensor responses in the lower extremity with the exception of the ankle and foot, which appear flaccid. An EMG of the leg and foot muscles detects abnormal resting potentials, including fibrillation and positive sharp waves in muscles innervated by the fibular nerve. Tapping on the muscles fails to elicit MUAPs. Muscles in the tibial nerve distribution have no resting potentials and respond with bursts of identifiable MUAPs when the tendon is tapped. These findings would guide the physician and therapist in looking for a possible peripheral nerve lesion in addition to the CNS dysfunction. The treatment program in this situation would differ from that for a patient without peripheral nerve pathology.
In summary, results of the needle EMG are best presented in the form of a table that indicates the following:
- •
Insertional activity: may be increased in acute denervation and myopathic processes
- •
Spontaneous activity at rest: present (positive sharp waves and fibrillation potentials) in denervation
- •
Fasciculations: may be present in motor neuron disorders
- •
Analysis of MUAPs:
- •
Amplitude: Low amplitude is seen in myopathy or nascent potential; large amplitude is a sign of chronicity.
- •
Phases: Normal MUAPs are biphasic or triphasic. Polyphasia is indicative of denervation-reinnervation.
- •
Recruitment pattern: A less-than-full recruitment is indicative of fewer motor units discharging, as can be seen in axon loss.
- •
Firing rate: If elevated, fewer motor units are contracting more often to provide the same tension in a denervated muscle.
- •
Justification and analysis of the basic principles and results after EMG studies should assist therapists managing patients with neurological dysfunction in planning and modifying therapeutic management programs. As electrical tests are being conducted, the findings are continuously studied and used by the physician and examiner as a guide in continuing with or modifying the plan for future tests based on whether they fit the characteristics usually identified with specific pathological conditions. As previously stated, the results of the electrodiagnostic tests must be correlated with other clinical findings and data.
Summary of clinical electroneuromyographic and nerve conduction studies
Instruments with computer-assisted analysis are now commonplace for studying electromyographic signals in great detail. , Parameters of the waveform, including amplitude, duration, frequency spectrum, number of turns, or phase polarity reversals and area (the integral or total voltage of the waveform), can be automatically analyzed. The data are then compared electronically with predetermined patterns of electrical changes, which correlate with categories of neuromuscular disorders such as myelopathies and neuropathies.
The following is a summary of the more characteristic EMG and nerve conduction changes associated with selected groupings of neurological disorders. The intent is to assist in the understanding of reports of these studies and recognize changes that may be seen in sequential tests during the course of the disorders. The following is a simplified grouping of electrical changes; actual electrodiagnostic studies show considerably more detail and frequent variations of these findings. , ,
Electrical testing in CNS disorders typically shows normal motor and sensory nerve conduction. In the EMG, spontaneous activity is typically not seen, and individual motor units seen on muscle contraction usually have normal parameters. The recruitment pattern may show a slower-than-normal MUAP discharge frequency with an incomplete and irregular interference pattern. In the presence of tremor and other involuntary movements, bursts of MUAPs occur, consistent with the muscle contraction pattern. In cases involving the brain stem, the blink reflex may show abnormalities. The tests are important in differential diagnosis between a CNS and a PNS problem, but often they are not used when clinical examinations demonstrate the problem to be definitively in the CNS.
In myelopathies, which include upper and lower motor neuron disorders (e.g., amyotrophic lateral sclerosis [ALS], poliomyelitis, cervical spondylitis, and syringomyelia), motor and sensory nerve conduction is usually normal, although mild slowing may be present. Motor amplitudes may be decreased. The characteristic EMG changes, which usually appear in the more chronic stages of the disorders, are increased amplitude and duration of MUAPs because of the variable impulse conduction time in sprouting axon terminals. An increased number of polyphasic potentials with increased duration is usually found. Spontaneous activity is often seen, and on strong contraction fewer rapidly firing large MUAPs are recruited, resulting in a single-unit or partial interference pattern. Fasciculations and denervation potentials are typically found in ALS. The distribution of the EMG abnormalities determines the extent of the condition.
Peripheral neuropathies show a variety of electrical changes depending on the type and location of the pathology. In a proximal pathology (e.g., radiculopathy), motor and sensory nerve conduction generally remain normal, except F waves and H-reflex responses in specific spinal segments. If motor nerve roots are compromised, spontaneous activity and increased polyphasic potentials appear, and reduced recruitment of MUAPs results in an incomplete interference pattern. In more chronic stages MUAP amplitude and duration can be increased. As the lesion improves, spontaneous activity decreases and the recruitment patterns become more normal. If only sensory roots are injured, no EMG changes occur. Again, the distribution of the EMG abnormalities (all in one myotome) is pathognomonic, especially in the presence of denervation potentials in the corresponding paraspinal muscles.
Lesions of peripheral nerves, which range from a focal mononeuropathy to plexopathy, frequently show abnormalities in motor and sensory nerve conduction, depending on which components of the nerve are involved. In the EMG, spontaneous activity, particularly fibrillation and positive sharp waves, is common. If the lesion is complete, no MUAPs are found. The presence of even a few MUAPs suggests a more optimistic prognosis. Often the location of the lesion can be identified by the distribution of the electrical changes. With regenerating axons, low-amplitude polyphasic MUAPs gradually appear. In the chronic stage, the amplitude and duration of MUAPs are often increased. Spontaneous activity decreases with reinnervation, but it may persist for several years.
Generalized, systemic peripheral polyradiculoneuropathies can be divided into primarily demyelinating, primarily axon loss, or mixed axonal-demyelinating polyneuropathies. Some involve mostly sensory nerves (e.g., hereditary sensory neuropathy types I to IV, Sjögren syndrome, Friedreich ataxia), and others mostly motor nerves (e.g., chronic inflammatory demyelinating polyneuropathy [CIDP], lead neuropathy), but most involve both sensory and motor nerves. In the primarily demyelinating type, such as Guillain-Barré syndrome, motor and sensory nerve conduction become markedly slow and F-wave latencies are delayed. EMG changes usually do not occur, except for a reduced recruitment pattern consistent with weak muscle contraction or conduction block (when the demyelination is such that the impulse does not propagate). With primarily axonal polyneuropathies, such as uremic neuropathy, isoniazid or cisplatin toxicity, and lead poisoning, motor and sensory nerve conduction is mildly slowed or may remain normal. The duration and amplitude of the response, however, decrease. During advanced stages, many polyneuropathies develop both demyelinating and axonal pathology (e.g., diabetic neuropathy, which is by far the most commonly encountered polyneuropathy). On EMG, spontaneous activity is commonly seen. These electrical changes generally become more severe with worsening of the pathology, but they also improve if the pathology is reversed. From a patient management standpoint, remyelination occurs at a much more expedient pace than reinnervation.
Again, the scope of the NCS electromyographic test is to determine the presence and extent of a neurological dysfunction. Attributing a cause to the dysfunction (e.g., diabetes versus alcoholism) requires other tests.
With myopathic disorders, motor and sensory nerve conductions are generally normal unless neural tissue is also affected. In advanced stages, however, severely atrophied muscles can produce decreased amplitude and distorted nerve conduction responses. The characteristic findings on EMG are short-duration, low-amplitude potentials. Some spontaneous potentials, particularly fibrillations and positive sharp waves, may be found but are much more frequent in the inflammatory myopathies such as polymyositis. Specific myotonic potentials appear in certain myopathic disorders (e.g., myotonia congenita). The recruitment pattern shows many low-amplitude MUAPs, appearing in a full pattern, with little voluntary effort. This type of recruitment pattern is referred to as early recruitment.
Neuromuscular junction disorders involve the synapse between axons and myocytes. In LEMS, the pathology is in the presynaptic membrane (decreased release of acetylcholine [ACh]), whereas, in myasthenia gravis, the pathology involves the postsynaptic membrane. The two are differentiated by the response to RNS or jitter with single-fiber EMG (see later). In LEMS, the amplitude of the responses increases with repetitive stimulation (more quanta of ACh released), whereas, in myasthenia gravis, the responses decrease (all ACh receptors saturated). Sensory NCS findings are typically normal. Table 31.1 provides a summary of typical findings.
Disorder | Motor Conduction | Sensory Conduction | Electromyography |
---|---|---|---|
Motor neuron disease (e.g., amyotrophic lateral sclerosis [ALS]) | Reduced amplitudes | Normal | Acute plus chronic neurogenic changes, fasciculations |
Radiculopathies | Normal | Normal | Acute neurogenic changes in myotome |
Plexopathies | Reduced amplitudes | Reduced amplitudes | Acute neurogenic changes in specific pattern |
Axonal neuropathy | Reduced amplitude in affected nerve(s) | Reduced amplitude in affected nerve(s) | Acute neurogenic changes in affected nerve |
Demyelinating neuropathy | Reduced conduction in affected nerve | Reduced conduction in affected nerve | Normal |
Neuromuscular junction disorder | Decrement (myasthenia gravis [MG]) or increment (Lambert-Eaton myasthenic syndrome [LEMS]) with repetitive stimulation | Normal | Occasional myopathic motor unit action potentials (MUAPs) |
Myopathies | Normal | Normal | Small-amplitude polyphasic MUAPs |
Single-fiber electromyography
Electrical activity can be recorded from two or more muscle fibers innervated by the same motor unit by using a specially designed single-fiber needle electrode. SFEMG is, at this time, the most sensitive test for evaluation of neuromuscular transmission defects such as myasthenia gravis and myasthenic syndrome. It is also used to evaluate peripheral neuropathies, motor neuron diseases, and myopathies. It is typically provided only in tertiary centers and requires a high level of patient participation.
During a carefully controlled minimal voluntary contraction, a 25 μm–diameter needle is inserted into the muscle, and several potentials from muscle fibers within the recording area are stored. Equipment with a trigger and delay line is necessary to “time lock” the tracings of the potentials. The slightly different conduction time or interpulse interval (IPI) required for impulses to be transmitted from a single motor neuron to each of its terminal endplates, cross the neuromuscular junction, and activate the muscle fiber is called jitter. This time difference is collected from several tracings and is converted into a mean consecutive time difference (MCD), which normally ranges from 5 to 55 msec. Values shorter or longer than this range are considered abnormal. The impulses from some axons to their muscle fibers may fail to be transmitted. This is referred to as blocking. Another capability of SFEMG is the measure of fiber density, that is, the average number of muscle fibers within the needle recording area. Fiber density is increased in reinnervation and also with certain myopathies because of axonal collateralization or splitting.
Macroelectromyography
A variation of SFEMG uses a macroelectrode to record the majority of muscle fibers of a single motor unit as they are triggered by an initial potential, which is then time locked with all the other muscle fiber potentials recorded from a different part of the same or a nearby needle. , , , , Two recording channels are used. Maximal amplitude of the potentials from several muscles has been reported by Stalberg. The findings are analyzed, along with findings of jitter, fiber density, and conventional EMG, to evaluate the status and prognosis of various neurological and neuromuscular disorders, such as motor neuron disease, peripheral nerve lesions, and myopathies.
In summary, the astute PT in the presence of a patient reporting weakness and/or numbness will refer that patient appropriately for electrophysiological testing based on the findings of a judicious clinical examination. In reading the report of an electrophysiological consultation, the PT will first correlate the results with the findings of the physical examination of the patient, determine whether the studies performed are complete (i.e., there are sufficient data to rule in the condition but also sufficient data to rule out other conditions), and correlate the findings with the conclusion. There is evidence that PTs performing NCSs and EMG tend to follow guidelines consistently.
Kinesiological electromyography
KEMG measures muscle activation during movement, whether it is purposeful, involuntary, dynamic, or relatively static. It is the method by which the therapist-examiner determines a muscle’s (or muscle group’s) onset, cessation, relative intensity, and activation sequencing during functional activities such as walking. Because normal movement depends on the CNS’s ability to execute motor programs through muscle action, KEMG provides the therapist with insight, in real time, into motor function, motor control, and motor learning.
Persons with neuromuscular disorders typically exhibit control errors, including the inability to initiate, execute, or terminate movement. Selective control may be absent or abnormal with errors in muscle timing, intensity, and sequencing. Spasticity or synergistic muscle action may impede smooth execution of tasks and prevent purposeful movement. With increased emphasis on evidence-based practice, KEMG can provide objective documentation of abnormal control and intervention outcomes and provide insight into optimizing strategies for improved functional performance. Many orthopedic surgeons rely on KEMG testing to supplement clinical evaluation in planning surgical interventions (muscle transfers and releases) in children with cerebral palsy (CP), in patients with TBI, and in those who have had a stroke.
KEMG interpretation depends on the examiner’s understanding of the instrumentation chosen for testing, including electrode selection, recording techniques, signal processing, and time and intensity normalization. Coupled with three-dimensional motion (infrared camera and motion capture) and force plate analysis, external moments are calculated that define internal force demands on muscles during functional activities such as walking. KEMG delineates the muscles that participate in meeting the internal force demand.
Recording instrumentation
KEMG can be performed by using surface or fine wire electrodes (intramuscular). Controversy exists about the choice of electrodes, with the selection dependent on the clinical or research question. If the examiner is interested in “muscle groups” (e.g., dorsiflexors, quadriceps), then surface electrodes are appropriate. Fine wire electrodes are optimal if activation of individual or deep muscles is desired (e.g., posterior tibialis, iliacus). Fine wire allows for specificity of muscle action required for surgical decisions related to muscle transfers, releases, or muscle lengthening. , ,
Needle or fine wire electrodes (indwelling or intramuscular).
A pair of 50-μg fine wire electrodes, also referred to as indwelling or intramuscular electrodes, are introduced through the skin and into the muscle with a 25-gauge hypodermic needle. The 50-μg, Teflon-coated wires are threaded through the needle’s core; 2 to 3 mm of the wire’s distal end are stripped of insulation and, once inserted, record adjacent motor unit activity. Before insertion the electrodes are sterilized. Inserted through the skin and into the muscle of interest, the barbed end “hooks” the muscle fibers when the needle is withdrawn. Accurate placement requires that the examiner have extensive knowledge of three-dimensional anatomy and excellent palpation skills. A maximal concentric voluntary contraction is elicited to anchor the wires into the muscle fibers, preventing displacement during subsequent contraction. This ensures sampling the same motor unit pool during subsequent tasks, trials, or conditions. ES is an essential testing element to verify electrode location. Wire electrodes allow a more precise definition of muscle timing (onset and cessation) by reducing the incidence of intramuscular crosstalk. Disadvantages include decreased reliability and insertional pain caused by skin penetration. In several states, the examiner must possess specialized KEMG licensure to penetrate the skin with a needle.
Surface electrodes.
When the clinical question can be answered by using surface electrodes, ES of motor points often defines optimal electrode placement over the muscle or group of muscles of interest. A maximal voluntary contraction (MVC) is elicited to confirm that optimal placement has been achieved. Standardizing electrode placement, size, interelectrode distance, and skin preparation enhances test-retest repeatability, with submaximal contractions being more reliable than maximal contractions. Skin displacement under the recording site may introduce movement artifact, which can be minimized by securing the electrodes to the skin with tape. To improve interday reliability, electrode placement should be marked (with ink) and standardized electrodes used. When both recording electrodes are contained in the same housing, interelectrode distance is standardized and movement artifact attenuated. Advantages of using surface electrodes include improved reliability and the ease with which they can be applied without causing patient discomfort. Specialized licensure is not required.
Instrumentation for kinesiological electromyography acquisition
KEMG signal acquisition requires either a telemetry unit (FM modulation) or a hard-wired system that relies on a “cable” tethered to the subject to transmit signals from the electrode site to the receiver. The subject’s performance and nature of movement strategies performed may be altered by the cabling. Telemetry allows the subject unrestricted movement; KEMG signals are transmitted through the air from a small unit worn around the subject’s waist. The optimal characteristics of the receiver include a bandwidth frequency of 40 to 1000 Hz and an overall gain of 1000 Hz.
Signal processing.
KEMG processing has become highly automated with the advent of high-speed computers and customized software. Once the signal has been acquired, it is stored digitally and processed by various computer programs. The “raw” signal is full wave rectified (all the negative values become positive), and a linear envelope is generated within a designated time interval. The area under the curve is mathematically integrated, and an average EMG profile is generated. Muscle-specific onset, cessation, and relative intensity are defined with a variety of software. According to a recent study, KEMG timing (onset and cessation) is optimally identified by using the intensity-filtered average (IFA) and packet analysis (PAC) when compared with ensemble average (EAV). Despite a smaller recording volume with wires, Bogey and colleagues demonstrated no significant difference in signal amplitude when multiple insertion sites within the same muscle were compared.
Normalization.
Any acquired “raw” EMG signal needs to be referenced to a standard value. This is accomplished by dividing the raw EMG during a functional task such as walking by a reference value. The MVC serves this purpose. Subjects exert a maximal voluntary effort for each muscle that determines the maximal EMG activity possible. All subsequent efforts are compared with this maximal effort and expressed as a percentage of maximum (%MVC). In patients with neurological dysfunction who lack selective motor control, a maximal effort can be elicited in either an extensor or flexor synergy by using the upright motor control (UMC) test developed at Rancho Los Amigos National Rehabilitation Center, grading the effort as “weak,” “moderate,” or “strong” in synergy. Maximal efforts are elicited for 3 to 5 seconds, and the software determines the maximal activity for a 1-second interval. The muscles’ activation during a functional activity is subsequently expressed as a percentage of MVC.
Interpretation of kinesiological electromyography
Kinesiological electromyography and strength.
KEMG testing does not directly measure muscle strength, and the examiner should resist equating raw EMG signal amplitude directly with muscle force or torque output. Grading the strength (manual muscle testing [MMT] or UMC test) of each maximal effort must accompany the interpretation of the muscle participation during a functional task. For example, patients with postpolio syndrome (refer to Chapter 35 ) produce large-amplitude KEMG signals that often reflect the maximal exertion of a “weak” muscle (e.g., “MMT—Poor” or ⅖). Large-amplitude EMG signals represent activation of large motor units typical of reinnervation, not force output. Despite large-amplitude signals, the muscle is functionally weak. In other words, a 100% MVC normalized KEMG record for a muscle may represent the maximal effort of a “poor” or ⅖ muscle.
Muscle tone versus spasticity.
Therapists should resist making inferences about tone from KEMG testing. As previously stated, KEMG reflects the contractile activity of motor units. Muscle tone refers to the amount of resting tension in a muscle because of its viscoelastic properties. Because tone is not a function of motor unit activity, it cannot be measured with KEMG. In contrast, spasticity, defined as a hyperactive quick stretch response, can be recorded by KEMG because it reflects prolonged muscle activation (Ôºû0.1 second). Clonus has a distinct frequency characterized by a prolonged 5- to 8-Hz signal. Using signal duration in response to quick stretch, Cahan and colleagues identified significant decreases in spasticity in selected lower-extremity muscles in children with CP after selective dorsal rhizotomy. In these children, spasticity interfered with agonist activation during walking.
In conclusion, KEMG is useful for delineating patterns of muscle activation in motor performance, reflecting the integrity of the neuromuscular control mechanism. The examiner’s interpretation should also consider additional factors, such as the type of contraction; speed of movement; joint acceleration; and a host of physiological, biomechanical, anatomical, and neurological elements beyond the scope of this chapter.
Electrical stimulation and electromyographic biofeedback
NMES and EMGBF are often used as tools in the management of neurological dysfunction. EMGBF can be used both alone and in conjunction with stimulation. The primary goal of use is improvement of function by improving voluntary motor control. To that end, strengthening and alteration of abnormal tone are also common goals of treatment. EMGBF is discussed later in this chapter. More detailed explication of treatment protocols is included in a variety of published work.
Electrical stimulators used in physical rehabilitation practice may be either small, portable, battery-operated units or larger line-powered clinical instruments. The clinical units often will offer a variety of stimulus forms and options for modulation of currents. Portable units provide the ability for patients and caregivers to carry out prescribed stimulation at home. Clinical units allow the therapist to customize programs to optimize treatment outcomes.
Neuromuscular electrical stimulation
NMES is often used as a tool in the management of neurological dysfunction. In NMES, muscle contraction is elicited by depolarization of the motor neurons. Electrodes may be placed over the muscle to be stimulated or over the motor nerve that controls the muscle. In NMES, muscle contraction is elicited by depolarization of the motor neurons. Electrodes may be placed over the muscle to be stimulated or over the motor nerve that controls the muscle. Firing order of neurons is a result of neuronal size, proximity of the electrical stimulus, and the intensity of stimulation. Muscle recruitment patterns triggered by ES differ from those observed in normal muscle activation. In a voluntary muscle contraction, motor units fire asynchronously, with a larger proportion of type I, fatigue-resistant muscle fibers of the smaller motor units being recruited first. The order of muscle fiber firing occurs as a result of motor neuron size and the anatomy of synaptic connections. Conversely, an electrically stimulated muscle contraction elicits initial responses from larger motor units, which contain a greater number of fatigable, type II muscle fibers. The type II fibers are innervated by larger-diameter neurons that have a lower threshold for ES than smaller neurons. A study of healthy subjects demonstrated recruitment of these higher-threshold motor units at relatively low NMES training levels. In voluntary exercise a much greater exercise intensity is required for activation of these larger motor units.
Synchronous recruitment of muscle fibers is obtained with ES. This does not occur with volitional activation. During a sustained volitional contraction, motor units periodically “drop out” and then “drop in” to reduce fatigue. With NMES, once recruited the motor units will continue to fire until the stimulus is ended. This, coupled with the early recruitment of fatigable motor units, accounts for fatigue being a major problem in the use of NMES. It also is one reason functional activities performed under control by stimulation are much less smooth and balanced than when they are performed volitionally. At the same time, relatively low levels of NMES can recruit motor units that volitionally would be recruited only with maximal effort. This provides support for observed increases in strength with low NMES training intensities. Numerous potential benefits have been identified for NMES, such as improvement in ROM, edema reduction, treatment of disuse atrophy, and improvement of muscle recruitment for muscle reeducation.
Parameters of stimulation
With any ES used for patients with neuromuscular dysfunction, three parameters must be considered within the waveform: pulse (or phase) duration, pulse frequency, and pulse amplitude. Depending on the intent of the ES, instruments are available that allow all of these to be independently adjusted.
Waveform
NMES units use alternating currents. The waveform of the stimulus produced by most NMES units is either a symmetrical or an asymmetrical biphasic pulse. The two phases of each pulse continually alternate in direction between positive and negative polarity. Although an ideal waveform has not been identified, most studies have shown the symmetrical biphasic waveform to be more comfortable than either the asymmetrical biphasic or the monophasic waveform. , ,
Duration
Phase or pulse duration (also called pulse width ) refers to the amount of time of a single pulse or phase. Stimulators with a pulse duration of 1 to 300 μs (0.3 ms) can be used to activate muscles with intact innervation. Waveforms of shorter durations require a greater current amplitude to produce a muscle contraction (because the current is on for a shorter period of time). They may be more comfortable but may not possess enough charge for good contraction levels. Longer-phase durations may be used but are less comfortable. In NMES units, pulse durations may be started at 100 μs, then increased to 200 to 300 μs if well tolerated by the patient. , Denervated muscles require significantly longer phase durations (20 to 100 msec) because of the longer chronaxy of muscle cells compared with motor neurons.
Frequency
Pulse frequency (or pulse rate ) refers to the number of electrical pulses applied per unit time. In applications seeking to provide muscle contraction, the stimulus rate should be 35 to 50 pps. This is a typical critical frequency at which a muscle will respond with a smooth contraction (also called a tetanic frequency ). Higher frequencies than this can result in early onset of fatigue. NMES used for spasm reduction may use a higher frequency, with the intent of fatiguing the muscle and decreasing spasm, which will result in decreased pain levels.
Amplitude
The amplitude (intensity) of the stimulus should be sufficient to achieve the desired strength of contraction. Battery-operated units usually indicate the amplitude only in a relative way (nonquantitatively) because the output of the battery declines over time. Depending on the impedance of the electrodes, coupling agent, skin, and soft tissue, the amount of current required at one location could be quite different than at another to produce the same degree of muscle contraction. This is why the amplitude of the stimulus is usually described according to the strength of the sensation felt by the patient. Mild, moderate, or maximum sensory refers to the intensity of the stimulation as felt by the patient, without eliciting a motor contraction. Similarly, mild, moderate, and maximum motor describe the amplitude of the stimulus needed to produce those visible muscular contractions using ES. If the intent is to strengthen the muscle or increase endurance, the patient is usually asked to participate by voluntarily contracting the muscle being stimulated when the stimulation is on. The amplitude of stimulation should be graded based on the response of the patient, aiming at production of the clinically desired force output.
Additional parameters: Ramp time and on-off time ratio
On time and off time.
NMES for facilitation of muscle contraction should be used to supplement exercise, and goals for stimulation should be consistent with the goals of the exercise program. To simulate isotonic or isometric muscle contractions, as in voluntary movement for exercise, the stimulator must have the capability of setting cycles of on and off times. Each period of muscle contraction is followed by a period of relaxation ( Fig. 31.7 ). , In most cases a shorter on time than off time is desirable to avoid fatigue. For example, a 10-second on time may be followed by a 50-second off time in a cycle, resulting in an on-off ratio of 1:5. Packman-Braun investigated ratios of stimulation to rest time with NMES for wrist extension in a group of hemiplegic patients. Results supported the on-off time of 1:5 as being the most beneficial in training programs of 20 to 30 minutes because of the deleterious effects of fatigue with lower ratios (1:1, 1:2, 1:3, 1:4). If the goal is to reduce edema by providing a muscle pumping action, a ratio of 1:1 or 1:2 may be preferred, as the intent is to decrease the edema by continuous muscle pumping action. Lower ratios may be used when the goal is neuromuscular reeducation or endurance training.

Ramping.
Ramping is another modulation that can be set by the therapist. Ramp-up is the time taken by each train of pulses to increase amplitude or intensity sequentially from zero to maximum. Ramp-off is the period set at the end of the train of maximal intensity pulses to decrease sequentially from maximum to zero amplitude (see Fig. 31.7 ). Ramp time can be adjusted so that the stimulation more nearly resembles a pattern of gradually contracting and relaxing muscles. For patients with hypertonicity or spasticity and a goal of facilitation and strengthening the antagonist muscle, a longer ramp-up time may avoid or minimize activation of the stretch reflex in the hyperactive agonist muscle.
Duty cycle.
The term duty cycle is sometimes confused with the on-off time ratio. Duty cycle is the percentage of time a series or train of pulses is on out of the total on and off time in a cycle. , For example, if the train of pulses is on 10 seconds and off 30 seconds, the total cycle time is 40 seconds. The duty cycle would be 25% (10 seconds of the total 40-second cycle). The actual on time and off time of the pulses in a cycle is a more informative description than either the duty cycle or the on-off ratio.
Muscle reeducation
After an insult or injury affecting the CNS, problems with motor control frequently manifest. One of the common goals of therapy is to facilitate movement in the areas where control is lacking. If active movement is not present, NMES allows movement to occur by stimulation, which may be followed by resumption of active movement, possibly triggered by the sensory (visual and proprioceptive, among others) experience that accompanies the stimulation. When active movement is present but is weak or not well controlled, the therapist may choose to use NMES to supplement and strengthen the muscular contraction already present. Some evidence exists that NMES can increase activity in the somatosensory cortex and that the cortical activity is correlated with improvement in functional tasks. In the presence of hypertonicity, the muscles serving as antagonists to the spastic muscle may be targeted for NMES, not only to strengthen the antagonist but to inhibit the spastic muscle by reciprocal inhibition.
Functional electrical stimulation
The term functional electrical stimulation (FES) has been used casually to describe various applications of NMES. However, FES is defined by the Electrotherapy Standards Committee of the Section on Clinical Electrophysiology of the American Physical Therapy Association as the use of NMES (on innervated muscles) for orthotic substitution. Baker and Parker use the term to describe external control of innervated, paretic, or paralytic muscles “to achieve functional and purposeful movements.” Although NMES is generally considered to have therapeutic applications, such as increasing ROM, facilitation of muscle activation, and muscle strengthening, the key to application of FES is to enhance or facilitate functional control. It is used with patients with SCI, TBI, cerebrovascular accident (CVA), and other CNS dysfunction who have intact peripheral innervation.
An example of FES application is the ES of the fibular nerve to enhance ankle dorsiflexion during gait in patients with hemiplegia. Two of the more common applications of FES on the market include the WalkAide system (Innovative Neurotronics, Austin, Texas) and Bioness (Bioness, Valencia, California). The L300 Go of Bioness (Bioness, Valencia, California) offers several features designed to optimize neurorehabilitation. This device uses a FES system with a three-dimensional motion detector of events in the gait cycle. Specifically, this device has a learning algorithm that analyzes the affected lower-extremity movement and induces ES directly to the phase of the gait cycle where it is required. Users of the earlier version (Legacy L300) have the tendency to be dependent on foot sensors or remote controls. This device has an adaptive motion detection feature and onboard controls to address this issue of dependency on foot sensors or remote controls. L300 Go also has a multichannel stimulation, which enables clinicians to adjust dorsiflexion and inversion/eversion with a novel new electrode options. To promote functional gains at home, a new mobile iOS application (myBioness) was developed to facilitate goal setting and tracking rehabilitation progress. In terms of neurological dysfunctions, research has been published that indicates efficacy of these devices and FES in general in improving functional performance in people with CVA, multiple sclerosis, TBI, SCI, , and CP. Moreover, numerous other uses of FES have been described, ranging from isolated motor control activities, such as decreasing shoulder subluxation and reducing scoliosis, to highly technical computerized gait and bicycling capabilities, sometimes referred to as computerized FES (CFES). The trigger that activates muscle contraction in synchrony with the functional activity can be manually initiated by the patient, set within the stimulator to automatically trigger on and off cycles, or programmed into a complex computer system for bicycling or gait.
Stimulation is generally applied in short-duration pulses with a frequency sufficient to provide smooth, tetanizing muscle contractions and adjusted to cycle on and off, with adequate ramp functions, as indicated by the speed and time needed to synchronize the stimulation with the functional activity. The length of the intervention depends on the purpose and may vary from a few contractions during the functional activity, building to multiple 30-minute sessions working up to several hours, repeated daily or three to five times per week. With the more complex computerized systems used for patients with complete spinal cord lesions, electrically activated functional movements are the mechanism to achieve physiological and psychological benefits. In some situations, assisted function is also an important goal, although functional community ambulation is not yet a reality. , , Hooker and co-workers evaluated the physiological effects of use of FES-assisted leg cycling in SCI. Compared with resting levels, significant increases were found in cardiac output, heart rate, stroke volume, respiratory exchange rate, pulmonary ventilation, and other physiological phenomena. CFES for cycle ergometry and ambulation has also been shown to increase muscle mass, electrically induce muscle strength and endurance, , , increase circulation and aerobic capacity, decrease edema, and have a beneficial impact on self-image. , , Jacobs and colleagues compared the metabolic stress of FES-assisted standing versus frame-supported standing. Cardiorespiratory stress was significantly higher with FES, and the authors concluded that FES-assisted standing alone may provide a stress sufficient to meet minimal requirements for exercise conditioning.
The demonstrated benefits of FES clearly indicate that it is a valuable tool for supplementing functional activities. The practicality and cost of applications of the more complex computerized systems need further study, especially in terms of function in community activities. (Refer to Chapter 38 for additional discussion.)
Electromyographic biofeedback
Biofeedback is a general term used to describe the use of visual or auditory representation of physiological processes to allow an individual to modify those processes. EMGBF makes available to the patient information regarding the electrical activity of muscle. EMGBF has several well-documented applications, including alteration of physiological responses such as heart rate, temperature, and muscle tension. These applications may prove beneficial for patients with neurological dysfunction. An example would be relaxation to modify pain perception. The focus of this review is the use of EMGBF for improvement of active movement, which may include reduction of hypertonicity in addition to muscle reeducation. EMGBF units range from basic single-channel portable models to clinical units with multiple channels and multiple options for provision of feedback.
EMGBF may be used to assist a patient in attaining greater levels of muscle activation in paretic muscle, decrease levels of muscle activation in spastic muscle, or attain a balance between agonist and antagonist muscle pairs. For most practicing clinicians, EMG levels are monitored through the use of surface electrodes. Monitoring of activation of deep muscles is often not feasible. Attention to size and specific electrode placement is critical to ensure feedback that will be useful. Smaller electrodes allow specific placement, although higher impedance will be encountered. Skin must be carefully prepared to take this into account. Because the EMG information recorded represents the sum of action potentials from motor units between the electrodes, large interelectrode distance will increase the area of muscle recorded. This may be desirable for large muscle groups or when minimal activity is present.
Smaller interelectrode distances are preferable if interference from “crosstalk” or “volume conduction” from muscles or motor units not part of the target group is a risk. Basmajian and Blumenstein provide an excellent review of electrode placements.
Reduction of hypertonicity
DeBacher described a progression of intervention with EMGBF designed to reduce spasticity. The program uses three stages of intervention: (1) relaxation of spastic muscles at rest even in the presence of distraction, mental effort, or use of muscles not targeted for EMGBF training; (2) inhibition of muscle activity during passive static and dynamic stretch of the spastic muscle, beginning with static stretch at the extremes of motion, then progressing to passive movement speed at a speed of 15 degrees per second; and (3) isometric contractions of the antagonist to the spastic muscle, with relaxation of the spastic muscle, progressing to prompt muscle contraction and relaxation of the spastic muscle, and grading of muscle contractions with movement for various force output requirements. Use of the technique in a small sample of young adults with CP demonstrated improvement in resting levels of involuntary muscle activity. Improvements in function, however, were not demonstrated.
Inhibition of a spastic muscle alone may not be enough to improve function. Often the spastic muscle itself, once hypertonicity has diminished, is weak or has low functional tone. Weak antagonists to the affected muscle may contribute to the functional limitation. EMGBF to reinforce activity in the weak muscle may be done concurrently with its use to modify the tone in the agonist. EMGBF can be useful in helping a patient decrease abnormal muscle activation, but persistence of control problems may be related to lack of force production, deficits in speed of muscle activation, and lack of reciprocal interaction of muscle groups.
Muscle reeducation
Therapists may opt to use biofeedback to provide information about the quality of the muscle contraction directly to the patient. The patient can then attempt to alter the contraction in accordance with guidelines provided by the therapist, whether the focus is to facilitate stronger contraction, decrease apparent hyperactivity, or modulate a balance of muscle activity during a functional task.
Concurrent assessment of muscle activity (CAMA) is an application of EMGBF in which the therapist uses biofeedback as an adjunct in evaluation of patient response to therapeutic exercise. In this procedure the therapist decides which muscle group(s) are desired for activation and adjusts the position of the patient or the therapist intervention accordingly to get the correct responses. CAMA allows for the judgment of the effectiveness of a particular activity based on actual EMG responses rather than presumptions of what the intervention should cause. In a placebo-controlled study of hemiplegic patients, the addition of EMGBF to hand exercises based on the Brunnstrom approach resulted in significant improvement in active ROM in those using biofeedback compared with sham.
Several authors suggest the use of biofeedback signals from homologous extremity muscles as a model for what the hemiplegic patient needs to alter muscle activity in a particular function. , This has been described as a “motor copy” and was compared with a more targeted training procedure. Indications showed that the motor copy resulted in better carryover in function than the comparison therapy in follow-up evaluations. A similar training study showed indications of benefits of the procedure, but the results were not statistically significant because of the small group size. At least two studies support patterning or copying EMG from other muscles as a potentially useful tool for individuals with C4 to 7 SCI. , A meta-analysis that compared EMGBF with conventional physical therapy for upper-extremity function in individuals after stroke reviewed only six studies and concluded that neither approach was superior to the other.
Feedback considerations
EMGBF has the benefit of being provided simultaneously with the patient’s movement, consisting of accurate and objective information about muscle activity (given careful electrode application), and not requiring the same level of therapist skill as verbal feedback provision. EMGBF therefore may be beneficial for patients with deficient sensory feedback systems. The frequency of feedback provision, however, may require close scrutiny by the therapist.
Experiments examining feedback frequency in the learning of motor tasks support the use of less than 100% relative frequency for the subject to learn the task. Feedback provided on every trial may improve performance but degrades learning in normal subjects. In a study of stroke patients attempting a pursuit tracking task, biofeedback was used for the experimental group (electrodes over the spastic biceps), whereas the control group performed the task without feedback. Posttests revealed that the use of continuous feedback had a negative transfer effect on learning of the movement task, suggesting that the experimental learners became dependent on the external feedback in performance of the task. The clinician must therefore carefully structure the use of external feedback so the patient begins to develop a sense of muscle activation or relaxation that is present without the EMGBF apparatus. This may be accomplished by turning the screen away from the patient and turning off the auditory signal as the patient progresses.
Integrating neuromuscular electrical stimulation and electromyographic biofeedback
The use of EMG-triggered NMES, in which NMES is initiated once the patient has achieved a predetermined level of EMG activity in the targeted muscles, is an application that has been shown to have merit, , although a more recent systematic review showed no statistically significant differences between EMG-NMES and usual care in improving upper-extremity function of the affected extremity in people who had had a stroke. Threshold levels of EMG activity could gradually be increased as the patient gains the ability to activate muscles independently, with eventual discontinuance of the NMES as strength and active control allow. The success of this application has been shown in patients with hemiplegia in terms of increasing EMG activity and subsequent improvement in ROM and function in the involved arm and leg. In another study, patients who had had a stroke more than 1 year previously significantly improved wrist and finger extension strength and function after treatment with EMG-triggered stimulation compared with controls.
A variation of this application is NMES triggered by positional feedback, such that NMES is initiated once the patient actively moves through a portion of the available ROM at a joint. The therapist may set the threshold angle in accordance with the patient’s goals and abilities. This method has been shown to be effective in improving wrist motion after stroke, although it was not as effective in altering control of the knee in a similar patient group.
Although discussions of EMGBF, NMES, and FES are often presented separately, the use of these modalities can be intertwined to achieve desired muscle control. NMES or FES may be initiated in the absence of active control (although lower levels of muscle activation may be discovered and facilitated with EMGBF). Once return of active control begins, EMGBF may be used to refine the control. An increase in muscle EMG should not be assumed to translate automatically to an improvement in functional use of that muscle in daily activities. Consequently, NMES and EMGBF need to be integrated into daily functional activities so that appropriate muscle activity is elicited and used in its appropriate functional context.
Applications
The application of the common principles of EMGBF and NMES to different patient populations emphasizes the role of the therapist in tailoring intervention to meet specific patient needs.
Many investigators have evaluated the use of NMES and EMGBF in patients who have had a stroke. , FES has been used extensively with patients with SCI. Other populations that demonstrate neuromuscular impairment or dysfunction have not been as thoroughly studied. This may be because the heterogeneity of these groups may create difficulty in research design.
Upper-extremity management
Electromyographic biofeedback
EMGBF has been extensively studied, but success of the treatment is mixed, with difficulty in interpretation. A reduction in co-contraction has been observed, as well as improvement in several neuromuscular variables , , ; however, a lack of significant improvement in functional skill was noted. Given the challenge of improving upper-extremity functional ability after stroke, Wolf and Binder-Macleod suggested consideration of several key factors in predicting which patients may benefit from EMGBF: “Those patients who achieve the most substantial improvement in manipulative abilities initially possess voluntary finger extension; comparatively greater active ROM about the shoulder, elbow, and wrist; and comparatively less hyperactivity in muscles usually considered as major contributors to the typical flexor synergy.” Attention to the chronicity of motor dysfunction also appears critical in anticipating success with EMGBF, as patients 2 to 3 months after stroke demonstrated stronger functional gains after intervention with biofeedback compared with patients 4 to 5 months after stroke. A placebo-controlled study of EMGBF showed statistically significant improvement in active ROM of the hand in patients who received EMGBF in addition to exercise. A study by Doğan-Aslan and colleagues found improvements in the Ashworth scale, Brunnstrom stage, upper-extremity function test, wrist extension ROM, and surface EMG potentials in their subjects who were treated with EMGBF in conjunction with neurodevelopmental therapy (NDT) and conventional treatment in a population of subjects with hemiplegia secondary to stroke.
Neuromuscular electrical stimulation
Common upper-extremity applications of NMES for the patient with a stroke include reduction of shoulder subluxation (FES) and facilitation of elbow, wrist, and finger extension and motor control.
FES for shoulder subluxation reduction appears beneficial for prevention of pain and subluxation, especially if used during the early stages of recovery. In 2018, Arya and his colleagues conducted a systematic review about rehabilitation methods for reducing shoulder subluxation in post-stroke hemiparesis. The researchers have reviewed 14 RCTs or controlled trials and 8 pre- and post-single group studies. In this study, researchers have indicated that FES is effective in reducing subluxation in acute stage and that shoulder support or orthosis may reduce the subluxation temporarily while in place. The functional benefits over the long term are not always clear, and cost-benefit ratios need to be considered. Baker and Parker, Faghri and colleagues, and Chantraine and colleagues have reported success in management of shoulder subluxation after stroke by using gradually increasing stimulation times that ultimately reached 6 to 7 hours per day. On-off ratios were typically 1:3.
Use of NMES after stroke to facilitate motor control and function has been studied, but many studies lack controls. The extensors of the fingers and wrist are typically the targeted muscle groups. de Kroon and colleagues reported a systematic review of literature that included six randomized controlled trials. A variety of stimulation parameters were used. Three studies tested subjects with acute conditions, and three tested subjects with chronic conditions. Outcome measures for motor control included the Fugl-Meyer Motor Assessment (four studies) and strength (two studies). Functional outcomes were reported by two studies, one using the Action Research Arm test and one using the Box and Block test. The authors concluded that there was a positive effect of stimulation on motor control. Only two studies reported functional outcomes, but both were positive. Of significance is the fact that only six studies met the criteria for review in terms of rigor. , , ,
FES has also been investigated as an upper-extremity orthosis after hemiplegia, using movement of the uninvolved shoulder to trigger stimulation of elbow extension and hand opening. After an extensive training period, patients were able to demonstrate functional use of the involved hand for basic reach and grasp.
Systems with more than two channels of stimulation have proved difficult for patients to use. Popovic and colleagues reported two studies in which they used NMES to treat patients after stroke. They termed the intervention functional electrical therapy (FET). The stimulation was used during an exercise program composed of voluntary arm movements and opening and closing, holding and releasing objects with the stimulation serving as an electric prosthesis. Treatment was 30 minutes daily for 3 weeks in one study and 6 months in the other. Outcomes were reported as better than controls. Initially higher-functioning subjects benefited more than those who were rated as low functioning. Unfortunately, outcome measures in these studies were not standardized.
A study of use of daily NMES in the form of neuroprosthetic FES (NESS Handmaster) stimulation of hand and finger extensors in patients with subacute strokes (6 to 12 months after onset) was reported by Ring and Rosenthal. All subjects were receiving physical and occupational therapy three times a week. The stimulator was used at home. Those receiving stimulation had significantly greater improvements in spasticity, active ROM, and functional hand test scores compared with control subjects. The authors concluded that supplementation of outpatient rehabilitation with NMES improves upper-limb outcomes.
Three-month interventions with EMG-triggered NMES, low-intensity NMES, proprioceptive neuromuscular facilitation (PNF) exercise, or no treatment were compared in a group of chronic stroke patients. At 3 and 9 months after treatment, Fugl-Meyer scores improved 18% for the PNF group, 25% for the patients receiving low-intensity NMES, and 42% for the group receiving EMG-triggered NMES. The control group did not change.
These findings lend support to the use of NMES and EMGBF and NMES alone as adjuncts to physical therapy. Typically, higher-functioning patients have shown the greatest impact. Results have tended to show greater effect in patients with acute or subacute conditions, but longer-term patients sometimes benefited as well.
With the advent of the use of technology in neurological rehabilitation (see Chapter 38 ), an increasing number of investigations have been done to incorporate new technology with ES to improve functional performance. Sayenko and colleagues described the use of a video game–based training system combined with NMES in a person with chronic SCI. Results indicated improvement in the strength and endurance of the paralyzed lower extremities of the individual after the intervention.
Lower-extremity management
Electromyographic biofeedback
Several studies evaluating EMGBF for retraining lower-extremity control after stroke have focused on improvement of tibialis anterior control and reduction of gastrocnemius muscle activity. Results support increases in strength and ROM in ankle dorsiflexion with carryover into ambulation and maintenance of this improvement on follow-up evaluation.
Wolf and Binder-Macleod examined a number of variables at the hip, knee, and ankle in a controlled group study of the effects of EMGBF. Subjects were assigned to one of four groups: lower-extremity EMGBF, upper-extremity EMGBF, general relaxation training, and no treatment. No significant changes were observed between experimental and control groups for EMG levels and ROM at the hip, but improvements were noted in knee and ankle active motion for the experimental group. Although subjects in the experimental group increased their gait speed, these changes were not significantly different from findings in the comparison groups.
Use of EMGBF with the intent of improving ambulation may require use of feedback during the task of ambulation instead of during static activity, as demonstrated by this study. Positional biofeedback regarding ankle position and traditional EMGBF were compared in a group of hemiplegic subjects. A computerized system provided audiovisual feedback during ambulation for both groups. Pretreatment and posttreatment measures of ankle motion, gait, and perceived exertion were conducted for the two treatment groups and a control group. The group receiving positional feedback increased walking speeds relative to the other groups, with improvements maintained at follow-up intervals of up to 3 months. The consideration of integrating feedback into functional ambulation bears further investigation.
Neuromuscular electrical stimulation
Fibular nerve stimulation has been documented as an assistance for patients with hemiplegia to improve ambulation. , Long-term stimulation with implanted electrodes has proved effective in improving gait patterns, but difficulties in achieving balanced dorsiflexion, infection, and equipment maintenance were drawbacks. ,
Shorter-term use of fibular nerve stimulation as an adjunct to traditional physical therapy may be considered. In a controlled study examining the use of 20 minutes of fibular nerve stimulation six times per week for 4 weeks, the stimulated group demonstrated dorsiflexion recovery three times greater than the control group, as measured by an average of 10 maximal dorsiflexion contractions. These improvements were regardless of site of lesion, age, or time since lesion. Surface electrode stimulation is effective, and gait parameters can be improved with its use. , If, however, a foot drop is the only major impediment to ambulation, lightweight plastic orthoses are a functional and much less expensive choice of intervention.
Multichannel ES has been used in the management of ambulation in patients who have had a stroke. , Although more effective than traditional gait training in some cases in terms of gait velocity and stride length, at follow-up evaluations 8 to 9 months after therapy the difference between groups had faded. However, the expense and availability of such systems make their use unlikely at this time.
NMES used for ankle dorsiflexion triggered by heel switch during gait and biofeedback to improve active recruitment of ankle dorsiflexors or relaxation of ankle plantar flexors has been studied in hemiplegic patients. Patients who received a combination of these interventions demonstrated significantly improved knee and ankle range parameters more rapidly than those using a single modality. This improvement was maintained over a 1-month period. Although all groups improved in gait cycle times, results in the combined intervention group were better. This may be attributable to the synergy of biofeedback and stimulation. Granat and colleagues also studied fibular muscle stimulation effects on gait parameters after stroke. After intervention the subjects showed significant control of eversion on all surfaces. The Barthel Index score also improved after intervention. However, no improvement occurred when the patients were not using the stimulator.
Evidence-based practice
Much of the literature evaluating the use of EMGBF and NMES involves patients with CVA. Although these studies have shown many significant results, interpretation of these findings in relation to what is recommended for clinical intervention is not as clear-cut. Improvements in generation of EMG activity and active movement are well documented, but the functional implications of these gains are not as well established. Clearly, in the current practice environment much of the focus is on function, so these techniques to improve muscle activation patterns must be put to functional use in the context of therapy. The therapist must consider the relevant factors that may predict success and critically evaluate outcomes during trial use of these modalities. Cost-benefit analyses must accompany any intervention using technology with the goal of regaining movement as quickly as possible and eliminating the use of equipment when practical.
Stroke
A number of studies have been published discussing the muscle recruitment problems observed after CVA. , , Knowledge of these problems is a prerequisite for determination of the appropriate application of NMES. Delayed recruitment of the agonist and antagonist is a relatively consistent finding. Other findings include delayed termination of muscle activity once initiated, presence of co-contraction of agonist and antagonist muscles, , lack of co-contraction, and maintenance of agonist muscle contractions.
Sander and colleagues found that NMES application to the tibialis anterior muscle for 30 minutes three times a week for 4 weeks was effective in improving strength of the affected lower extremity.
Howlett and colleagues investigated the effect of FES in improving activity after stroke, and whether FES is more effective than training alone in a systematic review with meta-analysis. Following predetermined search and selection criteria, researchers included randomized and controlled trials up to June 22, 2014. Eighteen trials (19 comparisons) were eligible for inclusion in the review. Results of the study showed that FES had a moderate effect on activity compared with no or placebo intervention. Furthermore, researchers also noted that FES had a large effect on upper-limb activity and a small effect on walking speed compared with control groups, and appeared to have a moderate improvement in activity compared with both no intervention and training alone. In the study, researchers suggested that FES should be used in stroke rehabilitation to improve the ability to perform activities.
Most recently, Biasiucci and colleagues demonstrated that brain-computer interfaces (BCI) coupled with FES can elicit significant lasting arm motor recovery in patients with chronic stroke. BCI translate brain signals into planned movements in patients with limb paralysis. BCI alone has an unclear efficacy and mechanisms as to motor recovery after stroke. In this study, patients who received brain-actuated functional electrical stimulation (BCI–FES) exhibit a significant arm functional recovery post intervention. The recovery lasted for 6 to 12 months after the termination of therapy. The results of the study demonstrated a promising BCI–FES combination therapy to facilitate functional neuroplasticity leading to a significant arm functional recovery patients with chronic stroke.
Lee, Lee, and colleagues compared virtual reality (VR) combined with FES with cyclic FES for improving upper extremity function and health-related quality of life in patients with chronic stroke in a pilot, randomized, single-blind, controlled trial. In the study, participants were patients 3 months post-stroke. A VR-based wearable rehabilitation device was used in combination with FES. This device was used during the virtual activity-based training for the intervention group while the control group received cyclic FES only. Both groups received 20 sessions over a 4-week period. Results of the study have shown that FES with VR-based rehabilitation may be more effective than cyclic FES in improving distal upper extremity gross motor performance post-stroke.
In a study done by Kuznetsov and colleagues, a tilt-table with stepping features combined with FES was applied to patients with hemiparetic ischemic stroke 3 to 6 days post-stroke. Rehabilitation may be more effective if started early; however, early training is frequently limited because of orthostatic reactions. The researchers’ goal is to prevent these adverse reactions through robotic tilt-table training plus FES (ROBO-FES). The researchers compared the safety and feasibility of ROBO-FES and robotic tilt-table training (ROBO) against the control, tilt-table training alone. There were no serious adverse events occurred during the study. They concluded that robotic tilt-table exercise with or without FES is safe and more effective in improving leg strength and cerebral blood flow than tilt table alone in this patient population. Hocoma has produced a device with a tilt table with a stepping movement functionality with (ErigoPro®) or without FES (Erigo®) (see Fig. 31.8 ).

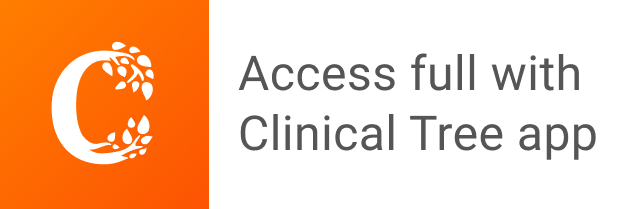