Fig. 4.1
Electrical stimulation is accomplished with simple square pulses, either monophasic or biphasic in nature. The pulses are described by their intensity (current) and pulse duration (time). The total charge is the product of the current and time. Symmetrical, biphasic pulses can be described as charge-balanced indicating that no net charge is delivered to the target tissue. The frequency of stimulation in Hz is the inverse of the interpulse interval
Chronaxie and Rheobase
Rapidly rising, square-wave pulses of either mono- or biphasic configuration can be described by their pulse duration and pulse amplitude. In order to understand the contributions of pulse duration and amplitude on the selectivity of neural stimulation, Lapicque undertook a series of investigations in the early 1900s using constant-current pulses. Lapicque described two concepts that illustrate the interaction between pulse duration and pulse amplitude and the excitability of neural tissues (Fig. 4.2). The first of these is rheobase which is the minimum stimulus amplitude needed to excite the target tissue, given an infinitely long pulse duration (practically speaking >10 ms). Lapicque further discovered that doubling of the pulse amplitude from its rheobasic intensity greatly diminished the stimulus pulse duration necessary to excite a given tissue, thus reducing the total energy necessary to achieve excitation. The pulse duration at twice the rheobasic strength he coined as chronaxie. It has been shown that chronaxie may vary across neural tissues. While most white matter tracts have chronaxie values in the 50–100 μs range, gray matter structures can have chronaxie values above 200–700 μs. As a practical matter, while stimulating in the periphery, a pulse duration of 100–300 μs is often chosen as the selectivity of neural structures is less important for these tasks. One exception to this is the recruitment of H-reflexes where pulse duration of 1,000 μs is typically selected in an attempt to preferentially recruit sensory fibers (see Chap. 12 on H-reflexes). When directly stimulating the cortex, the pulse duration is typically kept shorter (50–100 μs) in an attempt to avoid exciting cell bodies, which may lead to ictal activity.
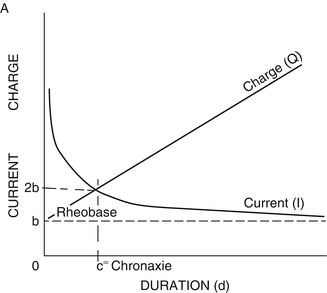
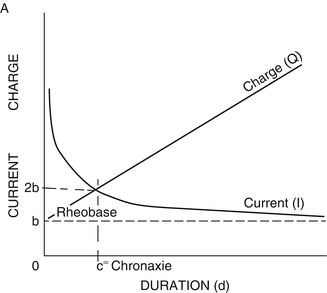
Fig. 4.2
Rheobase is the minimum stimulus amplitude needed to excite the target tissue, given an infinitely long pulse duration. Chronaxie is the pulse duration that excites neural tissue at twice the stimulus intensity of rheobase. This point also represents the lowest charge (Q, the product of stimulus intensity and pulse duration) at which the tissue can be excited
Constant Current vs Constant Voltage
Electrical stimulation can be applied in either a constant-current (current-regulated) or constant-voltage (voltage-regulated) manner. In the case of regulated voltage, the electromotive force (V) is held constant while the current (I) is allowed to fluctuate in response to changes in resistance (R), as dictated by Ohm’s law. As a result of this method of stimulation, the charge and electrical field will fluctuate as current varies in response to changing resistance. Fluctuating resistance will result from changing conditions at the electrode–tissue interface; for example, if an electrode is slightly repositioned or the composition of the conductive medium that surrounds the electrode is altered. As the charge and electrical fields vary between stimulus trials, the elicited response may become unstable. Voltage-regulated stimulation is susceptible to unstable responses due to this alteration of charge and electrical field. In the case of current-regulated stimulation, the current is held constant while voltage is allowed to fluctuate in response to changing resistance. The primary advantage of this configuration is that the charge and electrical field generated are more consistent, resulting in a more stable response. This makes current-regulated stimulation preferred for environments prone to rapid and extreme changes in resistance such as an open craniotomy.
Concluding Thoughts on Stimulation
It is important for the monitoring clinician to be able to understand the variable methods of electrical stimulation in order to appropriately apply current in a safe and effective manner. An understanding of the concepts discussed will give the monitoring clinician the tools to be able to reason through a stimulation task and select the appropriate stimulus parameters. We can, however, sum up with some general recommendations for common stimulation tasks. Most of the stimulation tasks in the surgical suite will utilize bipolar stimulation (most evoked potentials, somatosensory evoked potentials, direct nerve stimulation), with the most obvious exception being pedicle screw stimulation. Likewise, most of the pulses will be monophasic in configuration, with the most obvious exception being direct cortical stimulation for brain mapping. Pulse duration will be in the range of 100–300 μs except for H-reflexes (1,000 μs) and cortical mapping (<100 μs). Most tasks will employ a constant-current stimulation (pedicle screw stimulation, direct nerve stimulation, somatosensory evoked potentials, direct cortical stimulation using the Ojemann system), while others will employ constant-voltage stimulation (motor evoked potentials). For more specific recommendations, the reader is referred to the chapters contained within this volume for each modality.
Recording
Recordings of electrical responses can be made from tissues that generate or transmit electrical signals. This process generates differences in electrical potential between anatomic structures, which can be recorded by electrodes. Potential differences are generated by structures such as muscles, axons, and synapses that propagate electrical signals by the movement of ions across a membrane. Although a great many signals can be gleaned during an operation, those that relate to the integrity of underlying structures that might be injured during the procedure are identified for intraoperative monitoring. The presumption of intraoperative monitoring is that significant changes in the recorded signals represent potential injuries while preservation of baseline values should ensure that these structures and their associated functions are unaffected.
Recording Montages
Recording nomenclature can easily be confused with stimulation nomenclature. Most commonly, we make use of three montages or recording configurations. A referential recording is made whenever one electrode is placed over or near a generator of interest while a distant reference is placed in an electrically indifferent location such as over a bony prominence where little signal is expected. For instance, an electromyography (EMG) recording can be made from the medial gastrocnemius muscle with one electrode inserted over the belly of the muscle and a distant reference inserted over the medial malleolus. Such a configuration would have the advantage of sharing a large proportion of the ubiquitous electrical noise in common between the two channels, while not sharing much of the true signal of interest generated from the muscle. Referential montages are thought to give the largest signals; for this reason, they share little true signal in common between the two inputs, yielding little rejection of the true signal in the differential amplifier. A bipolar recording montage, not to be confused with bipolar stimulation, would be made if the distant electrode from the referential example above was moved from the medial malleolus to the medial gastrocnemius muscle. A bipolar recording is made when both recording electrodes are much closer together resulting in both electrodes recording some of the signal of interest. While less sensitive to activity generated at distances further from the electrodes, this configuration is highly specific. For this reason, bipolar recording is the most common montage employed in intraoperative EMG. The two adjacently placed inputs are ideally suited for recording the medial gastrocnemius muscle activity, although we can expect recordings of lower amplitude as some of their signals will be common to both inputs and thus be rejected by the differential amplifier. In practice, EMG signals are generally so large that a reduction of signal amplitude will be an acceptable trade-off when considered against the specificity advantage derived from the bipolar montage. Finally, we can also place needles in an active-referential montage by moving one of our needles to the lateral gastrocnemius muscle. By doing so we tie the medial and lateral gastrocnemius recordings together. The advantage of this configuration is that we can cover a larger number of muscles with fewer channels. When using modern intraoperative monitoring systems, this is often unnecessary as these newer machines have an ample number of channels (often 16–32) and there is no need to conserve channels for most procedures. The most likely exception would be a complex scoliosis procedure covering large areas of the spine and requiring monitoring from many muscles simultaneously. Should active-referential montages become necessary, the monitoring clinician must remember to combine muscles that arise from the same nerve root levels. For instance, creating a channel with one input over medial gastrocnemius, arising from the L5–S1 nerve roots, and another over tibialis anterior, arising from L4–L5 nerve roots, would be inappropriate as the monitoring clinician would be unable to accurately inform the surgical staff of the nerve root level at risk if EMG firing was noted from this channel. Similarly, a channel derived from the combination of biceps (C5–C6 nerve roots) and triceps (C6–C7) nerve roots would be inappropriate.
Volume Conduction
Volume conduction is the movement of charge through a conducting medium such as an ionic solution like salt water. This charge does not propagate in a directed manner; rather it radiates in all directions. As such it follows the inverse-square law along with other radiant phenomena such as light and sound. The inverse-square law states that the intensity of the signal will decrease with the square of distance. This radiant behavior means that a recording of a volume-conducted signal will weaken with distance in any direction from the signal source. As currents move through the body, they interact with recording electrodes, producing a potential difference between the electrode pair and resulting in a signal that can be recorded and interpreted. For example, summated signals from neuronal populations generate the electrical potentials recorded from scalp electrodes, with individual neurons having their own contribution to that population signal. If the individual neurons are arranged randomly, these potentials will cancel each other out, yielding no recordable signal. This is described as a closed electrical field and can be observed in the configuration of many brain nuclei. Fortunately, many of the signal generators of interest to neurosurgical procedures are arranged in an ordered manner, yielding an open electrical field and signals that may sum together and can be recorded at a distance. For example, pyramidal neurons of the neocortex are arranged in layers and columns. These neurons fire synchronously, resulting in a large potential that is oriented across the recording electrode and thus recordable.
With most recordings made in the surgical suite, it is impractical to place the recording electrodes directly in contact with the signal generator. As such, recorded signals are almost always transmitted to the electrode via some degree of volume conduction.
Understanding Current Sources
Although the reality is more complex, sources of current in the body can be modeled reasonably well by imagining that the body is a homogenous container filled with a conductive saline solution. In this situation a single current source will radiate current spherically with intensity that declines with the square of distance. This is referred to as a monopole. Potentials around this perfect monopole will be equivalent at equivalent distance from the current source. Any potential measurement made at a given distance from the monopole will be equivalent so long as radius from the source is kept constant. We can envision shells of equivalent potential strength, known as isopotential or equipotential field lines surrounding the monopole. However, true monopoles rarely exist. More commonly, current flows between two oppositely charged locations creating a dipole. A dipole consists of two oppositely charged areas, one a source and one a sink, with current flowing between them. Many of the cortical generators measured in the surgical suite can be modeled as dipoles. Dipoles generate fields of opposing polarity across their midline. Relative to an indifferent reference, a potential measurement on one side of the midline will be equal in strength but reversed in polarity from one made on the other side. At the midline the fields generated by the opposing poles of the dipole will cancel out creating a plane of zero potential strength. This feature can be taken advantage of when mapping the cerebral cortex as the plane of reversal corresponds to the central sulcus (Fig. 4.3).
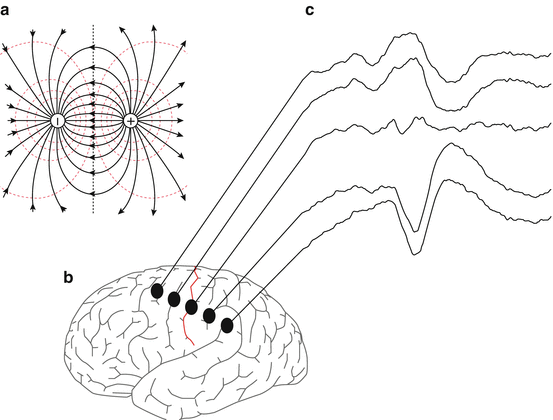
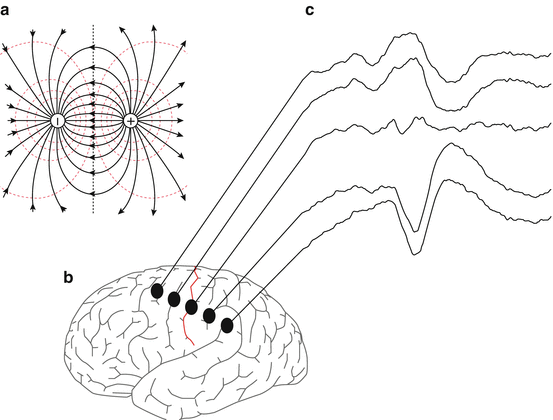
Fig. 4.3
An example of a perfect dipole (a), comprised of a current source and sink, and the field lines between them. The intersecting midline plane is an area of zero potential strength. Many neocortical generators can be modeled as current dipoles. An example is shown in (b) as the central sulcus divides the sensory areas (postcentral gyrus) from the motor strip (precentral gyrus). A grid of sensors can be laid across this strip, and evoked potentials can be recorded in response to sensory nerve stimulation (c). The reversal of phase from an upward deflection to a downward deflection in the recording represents the intersecting midline of zero potential generated from this structure. This can be used by the neurophysiological team to aid the surgeon in demarcating motor and sensory areas
Near- and Far-Field Recordings
Recordings are often referred to as either near or far field. Although these terms describe distance relative to an electrical field generator, the concepts are only indirectly related to the distance between the recording electrode and the electrical generator of interest. There are significant underlying biophysical properties that distinguish how near- and far-field recordings must be made in electrically noisy environments like the surgical suite.
Near-field potentials are generally recorded by electrodes placed directly on the field generator or in close proximity. Although these recordings are made in close proximity to the generator, they are still compound recordings or the sum of multiple potentials. Near-field potentials tend to be specific to a discrete neural structure. Near-field recordings can be either bipolar (closely located reference) or referential (distant reference).
Far-field potentials are those recorded at a distance from the generator. As such, they tend to record the contributions of multiple structures and can be less easily identified with a specific structure. The amplitudes of far-field potentials tend to be lower than those of near-field potentials, and they usually require signal averaging of multiple sweeps in order to detect the signal from extraneous background noise. When recording far-field potentials, both electrodes are relatively distant from the generator source.
Noise
In any observation of a physical phenomenon, there is bound to be some error in the course of measurement. This is known as measurement error and is defined as the difference between the actual or true score and the observed score of a phenomenon. This is a real but intangible concept as the degree to which a measurement is contaminated with error can never be known perfectly. When making electrophysiological recordings, we often refer to measurement error simply as “noise.” Ultimately, noise is any portion of the signal that may obscure the true signal. Sources of noise in the surgical suite can be varied, but one ubiquitous generator of noise emanates from electrical sources. In order to reduce the various noise components of our recorded signals, we employ methods including isolation of recording equipment from sources of noise, differential amplification, filtering, and signal averaging. The ultimate goal of all of these efforts is to maximize the signal-to-noise ratio (SNR).
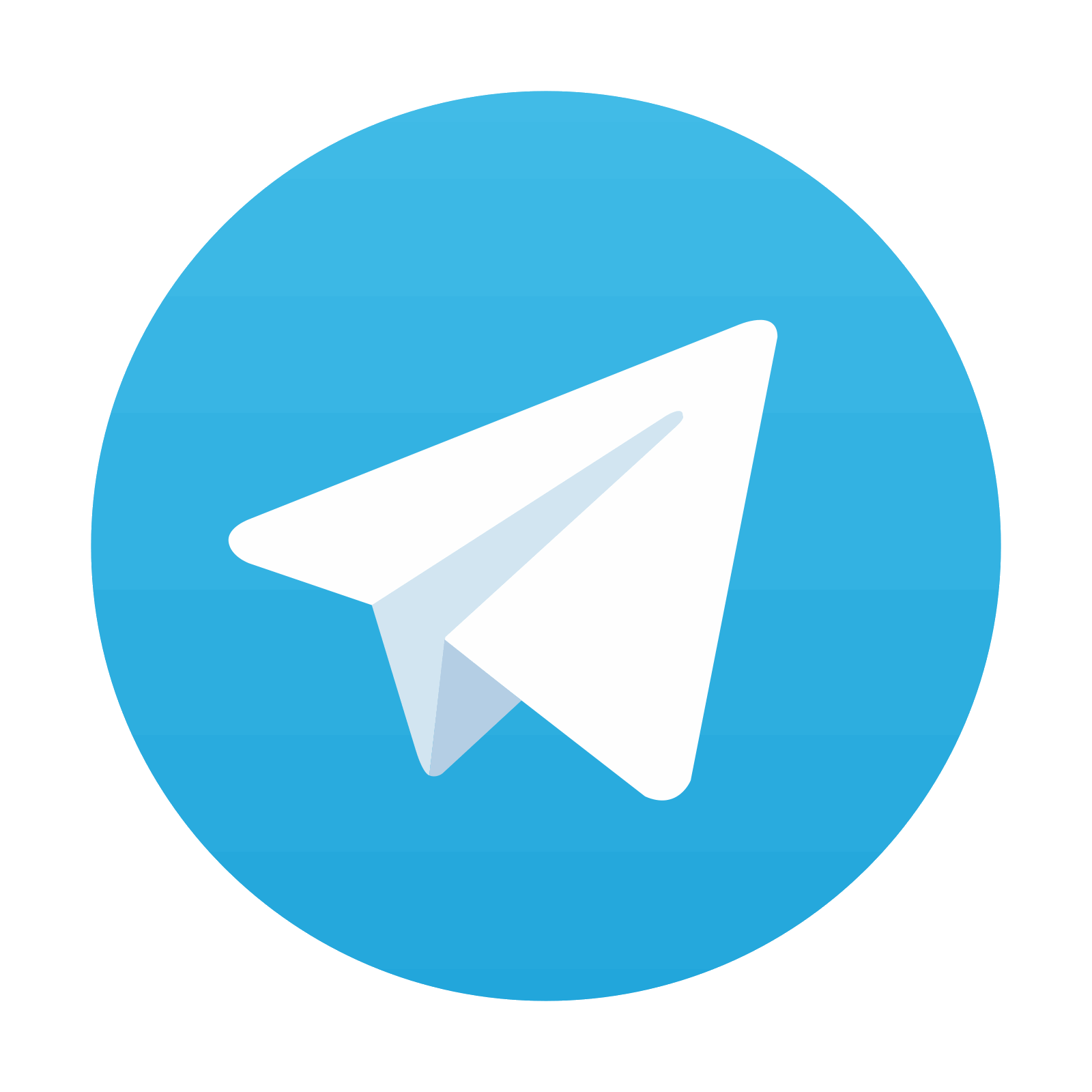
Stay updated, free articles. Join our Telegram channel
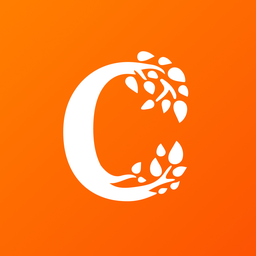
Full access? Get Clinical Tree
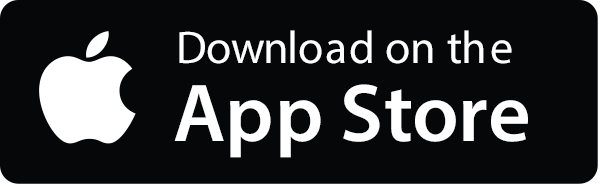
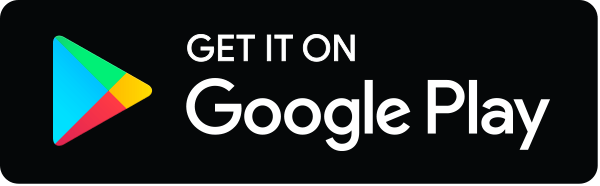