Abstract
The neuropathology of the encephalopathy of prematurity (see Chapter 14 ) includes injury to cerebral white matter and, in a major proportion of infants, a variety of neuronal-axonal disturbances. The encephalopathy includes a broad spectrum of initiating destructive events or disturbances in cellular maturation that are followed by widespread disruptions in cerebral white and gray matter growth and neuronal connectivity. This chapter emphasizes the pathophysiology of the most consistent and well-defined feature of the encephalopathy, injury to the cerebral white matter.
Keywords
oligodendrocyte, microglia, cerebral blood flow, maternal-fetal infection, inflammation
Overview of Pathogenesis
The preterm white matter is susceptible to a broad spectrum of injury severity that ranges from diffuse nondestructive lesions to the severe necrotic lesions of periventricular leukomalacia (PVL). The factors that contribute to the spectrum of severity remain incompletely defined, but a number of fundamental physiological factors related to cerebral blood flow (CBF), including cerebral oxygenation, hypercarbia, levels of glucose and its metabolites, as well as a variety of inflammatory factors, likely influence the severity of white matter injury (WMI). The propensity to injury is initiated by two major upstream mechanisms , primarily ischemia but also infection/inflammation ( Box 15.1 and Fig. 15.1 ). These mechanisms may operate in concert to potentiate each other. The critical downstream mechanisms involve at least three successive distinct injury responses that disrupt preterm white matter maturation at a critical period in development ( Fig. 15.2 ). The first coincides with early WMI and involves a confluence of maturation-dependent pathogenetic factors that selectively trigger the degeneration of late oligodendrocyte progenitors (preOLs) through hypoxia-ischemia and potentially other factors that include infection and inflammation. In response to preOL loss, early OL progenitors display remarkable plasticity, which is inherent to neuroglia. During this second subacute phase of WMI, early OL progenitors undergo a robust proliferative response that regenerates the preOLs required for OL differentiation and myelination. During the third and chronic phase of WMI, preOL differentiation is disrupted by the chronic injury environment, which coincides with disturbances in the normal progression of myelination.
a Includes those factors shown in human premature brain (see Chapter 13 for additional factors based on experimental studies).
Periventricular Vascular Anatomical and Physiological Factors
Potential arterial end zones and border zones
Very low physiological blood flow to cerebral white matter under basal conditions
Cerebral Ischemia, Impaired Cerebrovascular Autoregulation, and Pressure-Passive Cerebral Circulation
Danger of systemic hypotension
Danger of marked hypocarbia
Infection and Inflammation
Propensity for maternal/intrauterine infection and for fetal systemic inflammatory response
Propensity for postnatal infection
Presence of Toll-like receptors on microglia capable of producing pre-OL death on activation by release of ROS, RNS, and cytokines
Maturation-dependent concentration of microglia in cerebral white matter during the peak period of vulnerability to PVL
TNF-α in cerebrospinal fluid and brain in PVL
Interferon-gamma toxicity potentiated by TNF-α and greater to preOLs than to mature cells
Potentiating Relationship of Infection/Inflammation and Ischemia
Infection/inflammation leading to impaired cerebral perfusion
Microglia as a convergence point for both infection/inflammation and ischemia
Intrinsic Vulnerability of Cerebral White Matter of Premature Newborn
Vulnerability of preOLs to free radical attack
Production of both ROS and RNS
Deficient antioxidant defenses
Acquisition of iron
Vulnerability of preOLs to excitotoxicity
Exuberant expression of glutamate transporter
Exuberant expression of AMPA receptors, which also are deficient in the GluR2 subunit and therefore calcium permeable
Exuberant expression of NMDA receptors, which are calcium permeable
Presence of other potentially vulnerable, rapidly differentiating cellular elements
Axons
Maturation arrest of preOLs in chronic phase of white matter injury
Pronounced early proliferative response of oligodendrocyte progenitors precedes an increase in preOLs
Diffuse activation of microglia and astrocytes
Disruption of extracellular matrix signaling in lesions enriched in reactive astrocytes
Disturbances in normal progression of oligodendrocyte maturation and myelination
AMPA , Alpha-amino-3-hydroxy-5-methyl-4-isoxazolepropionic acid; NMDA , N -methyl- d -aspartate; pre-OLs , premyelinating oligodendrocytes; PVL , periventricular leukomalacia; RNS , reactive nitrogen species; ROS , reactive oxygen species; TNF , tumor necrosis factor.


Ischemia
Premature infants have a propensity for the development of global cerebral ischemia that particularly injures the white matter. Because of the limitations of current approaches to measure preterm human CBF, it has not been feasible to directly quantify human cerebral white matter flow to related disturbances in flow to WMI. Nevertheless, a role for ischemia is supported by a diverse array of clinical observations as well as experimental studies. Collectively, these findings support that moderately severe ischemia is necessary but not sufficient to generate WMI. The topography of WMI is related to a complex constellation of vascular anatomical, physiological, cellular maturational, and metabolic factors that define the timing of appearance and distribution of WMI.
Periventricular Vascular Anatomical and Physiological Factors
The development of the vasculature that supplies the preterm human cerebral white matter is significant for arterial end and border zones. Considerable study previously focused on the hypothesis that more severe focal necrotic white matter lesions coincide with regions of greater ischemia generated within these arterial end zones . (The earlier concept that the deep periventricular region also contained arterial border zones involving so-called ventriculofugal arteries is not supported by later studies as reviewed by Gilles and co-workers. ) The periventricular arterial end zones originate from vessels penetrating the cerebral wall from the pial surface. These long penetrating arteries are derived from the middle cerebral artery, and, to a lesser extent, the anterior or posterior cerebral artery, and they terminate in the deep periventricular white matter ( Fig. 15.3 ). The end zones that result are essentially distal fields in the periventricular white matter that are hypothesized to be more susceptible to a fall in perfusion pressure and CBF.

The penetrating cerebral arteries can be further divided into the long penetrators that terminate deep in periventricular white matter and the short penetrators that extend only into subcortical white matter (see Fig. 15.3 ). At 24 to 28 weeks of gestation, the long penetrators have relatively few side branches and infrequent intraparenchymal anastomoses with each other and with the short penetrators, which also are relatively sparse. Thus, end zones and border zones may exist at this time in the cerebral white matter relatively distant from the periventricular region. From 32 weeks to term, maturation of the short penetrators and the anastomoses between the long and short penetrators occurs and may promote CBF more similar to the term infant.
The susceptibility to hypoperfusion may also be influenced by the rate at which the vascular supply matures in the white matter. The last 16 weeks of human gestation is a period of active maturation of the periventricular vasculature. Indeed, this development could be used as an index of cerebrovascular maturity. Detailed analysis of the development of the vascular supply to the periventricular preterm white matter defined an avascular area at the common site of WMI. This avascular area may represent an area of vascular immaturity or an area of particular predilection to significant vascular necrosis that occurred in association with more severe WMI ( Fig. 15.4 ).

Consistent with the immaturity of the vascular supply of the preterm brain are human and experimental studies that have demonstrated the significantly lower basal blood flow to preterm cerebral white matter. Low basal white matter flow was suggested initially by xenon clearance studies that documented mean global CBF values in ventilated human premature infants of only ~10 to 12 mL/100 g/min. Subsequent xenon studies confirmed these very low mean global values (see Chapter 13 ). Importantly, studies of regional CBF by positron emission tomography confirmed the low global values and further documented that surviving preterm infants with normal or nearly normal neurological outcome had basal flow that ranged from only 1.6 to 3.0 mL/100 g per minute. These very low values in white matter were approximately 25% of those in cortical gray matter, a regional difference confirmed in a study using single photon emission tomography. These basal flow values of less than 5.0 mL/100 g per minute are markedly less than the threshold value for viability in adult human brain of 10 mL/100 g per minute ( normal CBF in the adult is ≈50 mL/100 g per minute). The very low values of volemic flow in cerebral white matter in the human premature infant suggest a minimal margin of safety for blood flow to cerebral white matter in such infants and suggests that the susceptibility of the preterm infant to WMI is related to a heightened propensity for ischemia.
Cerebral Ischemia, Impaired Cerebrovascular Autoregulation, and Pressure-Passive Cerebral Circulation
An apparent impairment of cerebrovascular regulation in sick premature infants may render cerebral white matter more vulnerable to injury from ischemia (see Box 15.1 ). Clinically stable premature infants seem less likely to exhibit this apparent lack of cerebrovascular autoregulation. Early radioactive xenon clearance studies showed that certain premature infants, mechanically ventilated and often clinically unstable, appeared to exhibit pressure-passive cerebral circulation ( Fig. 15.5 ). This fundamental observation has been confirmed by less invasive methods multiple times. Thus, in such sick premature infants with a pressure-passive cerebral circulation, it would be expected that when blood pressure falls, as occurs commonly in such infants, so would CBF, with consequent cerebral ischemia. With intact cerebrovascular autoregulation, CBF is not pressure passive but rather remains constant over a wide range of blood pressure because of arteriolar dilation with decreases in blood pressure and arteriolar constriction with increases in blood pressure (see the curve for the mature child in Fig. 15.6 ). The propensity to a pressure-passive abnormality in premature infants may relate in part to an absent muscularis around penetrating cerebral arteries and arterioles in the third trimester in the human brain.


The proportion of infants with a pressure-passive cerebral circulation and the duration of the abnormality appear to be substantial (see Chapter 13 ). In a serial study of 32 mechanically ventilated premature infants from the first hours of life, near-infrared spectroscopy (NIRS) was used to demonstrate a pressure-passive cerebral circulation in 53% (see Chapter 13 ). The nadirs of blood pressure often are not markedly low and thus could be readily overlooked with routine monitoring. Importantly, nearly all the cases of WMI (and severe intraventricular hemorrhage) were in the pressure-passive group.
In a later, more detailed study of a larger number of infants ( N = 90), pressure-passive periods were identified in 95% of the infants, and the overall mean proportion of the pressure-passive time was 20%. Some infants had a pressure-passive circulation more than 50% of the time. The likelihood of a pressure-passive state increased with decreasing gestational age and periods of hypotension.
Although the numbers were small, these studies suggest that (1) infants with impaired cerebrovascular autoregulation and a pressure-passive cerebral circulation could be identified by NIRS before the occurrence of WMI, (2) the circulatory abnormality was related to the occurrence of such injury, and (3) if the pressure-passive state could be corrected, the WMI could be prevented. A limitation of these studies is a lack of regional estimates of white matter flow . NIRS is limited to measurements of CBF at the cortical surface that may not reflect white matter flow during a suspected ischemic event. It would be particularly informative to measure temporal changes in white matter flow under basal conditions as well as during ischemia and reperfusion, as is feasible experimentally (see later).
Factors That Predispose to a Pressure Passive Circulation
A number of metabolic abnormalities that influence vascular reactivity are likely to promote the pressure passive state. Both hypocarbia (PCO 2 < 30 mm Hg) and hypercarbia (PCO 2 > 55 mm Hg) can significantly perturb CBF. Hypocarbia may promote hypotension and an increased risk for intraventricular hemorrhage and WMI through disturbances in CBF. The pronounced reduction in more severe cystic WMI appeared to be related to a decrease in days of mechanical ventilation, possibly related to reduced hypocarbic alkalosis, which can promote hypotension. Early studies identified that hypocarbia is a potential risk factor for more severe WMI. Analysis of the cumulative exposure to hypocarbia demonstrated an increased risk for more severe WMI that was confirmed in a large study of nearly 800 infants studied in the first 7 days of life and found to have a strong association with WMI identified as echolucencies on cranial ultrasonography ( Fig. 15.7 ). Infants with the highest quartile of cumulative exposure to hypocarbia had more than a fivefold increased risk of WMI when compared with infants in the lowest quartile. The contribution of hypercarbia to WMI is less well defined. Although trials of permissive hypercapnia found no evidence of cystic WMI by cranial ultrasound evaluation, abnormal white matter microstructure was identified when extremely low birth weight (ELBW) infants were evaluated at term by diffusion tensor magnetic resonance imaging (MRI).

Similarly unclear is the role of hypoxemia in promoting WMI through disturbances in cerebral autoregulation. Isolated acute hypoxemia has not been directly demonstrated to promote WMI independently of ischemia. Although transient hypoxemia caused inconsistent white and gray matter injury in the midgestation or near-term sheep, WMI was notably more severe when significant hypotension was observed.
Additional potential reasons for a pressure-passive cerebral circulation include the mechanical trauma of labor or vaginal delivery to the easily deformed cranium of the premature infant A combination of these factors or other factors (e.g., cytokine-mediated vascular effects) could be operative. Finally, even in the presence of intact cerebrovascular autoregulation , marked cerebral vasoconstriction or severe systemic hypotension could lead to sufficiently impaired CBF to vascular end zones and border zones to result in cerebral WMI. This explanation may account for the demonstrated relationships between marked hypocarbia or hypotension and WMI.
Factors That Influence the Timing and Distribution of White Matter Injury
A complex interplay of factors appears to define the enhanced propensity of the preterm human white matter to injury. As discussed earlier, these include an immature vascular supply and disturbances in cerebrovascular autoregulation that can particularly render CBF pressure passive in the setting of critical illness. Central unresolved questions are the factors that define the distribution of WMI and the developmental window of heightened vulnerability. Moreover, is a greater magnitude of ischemia necessary and sufficient to render certain regions more susceptible to more severe necrotic WMI? Often, but not exclusively, the focal necrotic lesions that affect all cellular elements localize to deep cerebral white matter in a periventricular distribution. These lesions frequently coincide with more superficial diffuse cerebral WMI that principally targets oligodendroglial precursor cells (preOLs). Is selective preOL death and the relative sparing of other cellular elements perhaps related to less severe ischemia sustained by diffuse WMI?
Multiple studies in preterm fetal sheep have confirmed that, as observed in humans, the cerebral white matter has an intrinsically lower basal CBF than other gray matter regions and also displays a pressure passive circulation relative to term and adult animals. We achieved spatially resolved quantitative measurements of fetal CBF in utero that were co-registered with histologically defined analyses of WMI. Flow in periventricular white matter was 60% to 70% lower than that observed in cerebral cortex. However, under conditions of moderately severe global cerebral ischemia, the nadir of ischemic CBF was similar in multiple cerebral gray matter regions relative to the white matter. After 30 minutes of ischemia, both cortical and white matter flows decreased proportionally to ~10% to 15% of basal flows. Later studies confirmed these observations and found that there were no pathologically significant gradients of flow between fetal cerebral cortex and deep cerebral white matter during either ischemia or reperfusion. Moreover, histologically confirmed WMI did not localize to regions susceptible to greater ischemia, nor did less vulnerable regions of white matter have greater blood flow during ischemia. These findings supported that ischemia is necessary but not sufficient to generate WMI ( Box 15.2 ). Even under conditions of moderately severe uniform ischemia, some regions of white matter were relatively spared, whereas other neighboring regions sustained significantly more cell death.
Basal flow was ~60%–70% lower in cerebral white matter than adjacent gray matter structures such as cerebral cortex and basal ganglia
During moderately severe ischemia, CBF decreased proportionally to ~10%–15% of basal flow in both white and gray matter
No measurable gradients of CBF between superficial cerebral cortex and deep cerebral white matter
Vulnerable white matter regions did not sustain greater reductions in CBF and blood flow was not greater in regions that lacked white matter injury
In regions with similar ischemic flow, the distribution of white matter injury was defined by the density of susceptible preOLs
CBF , Cerebral blood flow; preOL , pre-oligodendrocyte.
a See references and .
To address this paradox, in a series of studies in preterm fetal sheep we identified that in regions that sustained similar ischemic CBF, the distribution of WMI was explained by the distribution of one particular cell type, the preOLs (see Chapter 13 , Chapter 14 ). Human preOLs are markedly more susceptible to hypoxia-ischemia and oxidative stress than other neural cell types. In regions that contained more differentiated oligodendrocytes, the susceptibility to WMI was significantly reduced. The timing of appearance of preOLs during white matter development also significantly influences the magnitude and distribution of WMI. In preterm fetal rabbits, we identified a window in preterm white matter development (embryonic day 25) when the white matter is populated by a less mature population of early oligodendrocyte progenitors. These early progenitors give rise to preOLs and are much more resistant to hypoxia-ischemia than are the preOLs. Importantly, these early progenitors rapidly respond to injury by mounting a robust proliferative response that initiates repair of WMI by regenerating preOLs (see later). Consistent with these observations, the rabbit E25 white matter was highly resistant to pronounced cerebral ischemia in contrast to the E27 white matter when preOLs are diffusely present. Hence, both the timing of appearance and the distribution of preOLs define the location of susceptible regions of white matter under conditions of moderately severe ischemia .
It is also clinically important to identify the factors that define the pathogenesis of more severe WMI. The duration of cerebral ischemia is a critical factor that defines the burden of white matter necrosis. In preterm fetal sheep, graded WMI is generated that is related to the duration of ischemia. As discussed earlier, it is also likely that the magnitude of a number of systemic factors (e.g., hypotension) and metabolic factors (e.g., hypoxemia, hypocapnia, hypoglycemia, lactic acidosis) interact to determine the severity of ischemic WMI. Given how low basal CBF is in human preterm white matter, regional blood flow and metabolism may be equivalently low and matched for metabolic requirements. Ischemia may be relatively well tolerated unless there is pronounced energy failure that shifts the balance toward focal or more diffuse necrotic cell death. Hence, ischemia appears to be necessary but not sufficient to cause WMI, and cellular maturational and metabolic factors likely influence the severity of the WMI response .
It is also currently unclear if recurrent hypoxia-ischemia predisposes to more severe WMI. In preterm-equivalent neonatal rats, a pronounced increase in cell death was observed in chronic WMI after recurrent hypoxia-ischemia. However, in preterm fetal sheep that develop WMI that more closely resembles human, recurrent hypoxia-ischemia did not trigger more pronounced WMI. This suggests that recurrent hypoxia-ischemia may confer protection against more severe WMI through an ischemic tolerance-like mechanism. This is an important direction for future study.
Clinical Factors Supporting a Role for Hypoxia-Ischemia in the Pathogenesis of White Matter Injury
Additional clinical evidence supportive of a relationship between impaired CBF and the occurrence of WMI includes the association of the injury with markers of hypoxic-ischemic events (e.g., neonatal acidosis, elevations of plasma uric acid on the first day of life), episodes of mean arterial blood pressure less than 30 mm Hg, hypovolemia, oliguria, abrupt decreases in blood pressure in chronically hypertensive premature newborns (in whom cerebrovascular autoregulation may be present but with the curve shifted to the right), patent ductus arteriosus, congenital heart disease, and severely ill infants treated with extracorporeal membrane oxygenation (ECMO) or cardiac surgery. The adverse effect of patent ductus arteriosus on the cerebral circulation, documented by earlier studies, as well as subsequently, supports a direct link between cerebral ischemia and WMI. Thus, infants with the most severe derangement of CBF (i.e., retrograde flow in diastole) had a 36% incidence of PVL (ultrasonographic criteria) versus a 15% incidence in those infants with no retrograde flow. Escalating doses of indomethacin were not shown to reduce the incidence of WMI or intraventricular hemorrhage. The high frequency of the lesion in the hypoxemic and hypotensive infants treated with ECMO also demonstrates a striking relationship with apparent cerebral ischemia ( Table 15.1 ). Other pathological evidence for generalized ischemic insults (e.g., selective neuronal necrosis) also was present in these infants (see Table 15.1 ).
LESION | NO. (PERCENTAGE OF TOTAL) b |
---|---|
Periventricular leukomalacia | 18 (78%) |
Diffuse white matter injury | 8 (35%) |
Selective neuronal necrosis | |
Thalamus | 9 (39%) |
Inferior olivary nucleus | 8 (35%) |
Cerebral infarction c | 7 (30%) |
a Derived from 23 infants (born at 35 to 41 weeks of gestation) treated with extracorporeal membrane oxygenation at Boston Children’s Hospital from 1984 to 1991 (Kupsky W, personal communication, 1992). Nine infants (39%) also sustained subdural, subarachnoid, or intraparenchymal hemorrhage (see Chapter 22 ).
b Infants often had more than one lesion; thus, percentages exceed 100%.
A relationship of cerebral ischemia with WMI may be operative in infants with apneic spells. A relationship of apnea and bradycardia with WMI has been suspected for many years because earlier work, performed before the era of cranial ultrasonography, showed an association between spastic diplegia and apneic-bradycardic episodes. Studies of CBF velocity by Doppler showed an impairment in cerebral flow velocity with mild to moderate bradycardia (80 to 100 beats/min) and a marked impairment with severe bradycardia (<80 beats/min) ( Fig. 15.8 ). Studies with NIRS documented a sharp decrease in cerebral oxygenated hemoglobin and cerebral blood volume in association with apneic spells with bradycardia, and findings were consistent with the likelihood of a decrease in CBF with such spells. Chronic hypoxemia in rodents generates transient maturational disturbances in white matter myelination. Preclinical studies found that resolution of this dysmyelination was promoted by caffeine. Since caffeine therapy is an effective treatment for apnea of prematurity, its potential benefits to reduce WMI and related motor deficits were evaluated in several large clinical studies. However, longer-term outcome studies have not demonstrated reduced neurological morbidity related to caffeine therapy.

Several neuroimaging and postmortem studies have identified that near-term and term infants with congenital heart disease have reduced brain volumes associated with a high risk for WMI and that the WMI often precedes surgical interventions. In a large autopsy series of infants dying after cardiac surgery, WMI was the dominant lesion (nearly 80% of cases). Although WMI was originally thought to be related to perioperative hypoxia-ischemia during cardiac repair, more recent studies found that infants with congenital heart disease have low preoperative CBF that is associated with an increased risk for WMI. Reduced cerebral flow is associated with reduced cerebral oxygen delivery and reduced brain volumes. Survivors of cyanotic congenital heart lesions, particularly those with transpositions of the great arteries or hypoplastic left heart syndrome, are now surviving with significant motor and neurobehavioral disabilities that point to widely distributed white matter and gray matter disturbances that disrupt brain connectivity. The similarities between the pathogenesis of these conditions and preterm survivors are striking and support that disturbances in CBF and metabolism are linked to the spectrum of white matter and gray matter pathology in both disorders.
It should finally be emphasized that preterm infants and infants with congenital heart disease are also commonly at risk for exposure to hypoxemia or hyperoxia . The extent to which either insult independently contributes to WMI is currently unclear. Although exposure to both hypoxemia and hyperoxia is common in critically ill neonates, these infants are at risk for concomitant hypoxia-ischemia and other causes of WMI . It has thus been difficult in human studies to identify infants with relatively isolated exposure to hyperoxia. Similarly, there are no well-defined human neuropathological features that characterize the cerebral response to isolated chronic hypoxemia, because preterm survivors with chronic lung disease often sustain other forms of WMI related to hypoxia-ischemia, hyperoxia, or intraventricular hemorrhagic infarction. There are thus no human pathological studies that have defined the features of cerebral injury related to hyperoxia or hypoxemia, alone.
As recently reviewed and summarized in Box 15.3 , experimental findings from animal models have, in fact, identified that exposure to hypoxia-ischemia, hypoxia, and hyperoxia results in strikingly different forms of WMI. Global cerebral hypoxia-ischemia models in preterm fetal sheep, for example, display disturbances in cerebral autoregulation and regional white matter blood flow. These models generate graded cerebral WMI that closely resembles the spectrum of human preterm WMI that ranges from diffuse WMI to focal cystic necrotic lesions (see earlier discussion). In addition, acute WMI selectively triggers the necrotic and apoptotic degeneration of preOLs and results in chronic persistent reactive astrogliosis and microgliosis. By contrast, chronic hypoxia in neonatal rodents spares preOLs but causes delayed apoptosis of mature oligodendrocytes. Chronic hyperoxia similarly spares preOLs but causes apoptosis of early oligodendrocytes, which are the precursors to preOLs.
Potential Sources of CNS Inflammation Related to Systemic or CNS Cytokines
- ◆
Activated astrocytes and microglia are diffusely present in human WMI and adjacent peri-lesion white matter and represent a potential source of inflammatory cytokines in the CNS
- ◆
Elevated blood or CSF levels of TNF-α or INF-γ in preterm human infants
- ◆
TNF-α and IL1-β are detected in human autopsy cases with early WMI
- ◆
INF-γ localizes to reactive astrocytes in human autopsy cases with chronic WMI
- ◆
Circulating IL1-β and IL-6 cross the blood-brain barrier to access the CNS in preterm fetal sheep, providing a route for systemic cytokines to access cerebral white matter
- ◆
Ischemia-induced disruptions in the blood-brain barrier in preterm fetal sheep are attenuated with neutralizing antibodies against IL1-β and IL-6
Toxicity of Inflammatory Cytokines to CNS White Matter in Experimental Models
- ◆
Maternal exposure to bacterial endotoxin may cause WMI via vasoactive mechanisms that cause fetal cerebral ischemia or via stimulation of systemic cytokines that cross the fetal blood-brain barrier
- ◆
Maternal endotoxin exposure increases fetal CNS microglial activation
- ◆
Microglial derived TNF-α and INF-γ synergistically interact to cause early preOL death via nitric oxide-mediated toxicity or delayed death via cytotoxic cytokines, including TNF-α
- ◆
Exogenous exposure to IL-1β disrupts oligodendrocyte maturation and myelination
IL1-β, Interleukin 1-β; INF-γ, interferon-γ; TNF-α, tumor necrosis factor-α; WMI, white matter injury.
Although neither hypoxemia nor hyperoxia generates a spectrum of pathology that resembles human WMI, improved animal models are clearly needed to further define how hypoxemia or hyperoxia modifies the response to hypoxia-ischemic to disrupt cerebral white or gray matter maturation. Isolated chronic hypoxia, for example, disrupts EGF receptor-mediated mechanisms that are required for the survival and generation of new oligodendrocytes and thus may have significant consequences for white matter regeneration and repair processes. Moreover, chronic hypoxia disrupts preOL maturation and myelination through dysregulation of Wnt activity. Oxygen tension via hypoxia-inducible factor also influences Wnt activation to promote preOL maturation arrest and disrupts white matter angiogenesis, which is required for axon integrity as well as maintenance of neural stem cell niches for neurogenesis.
Infection/Inflammation
Infection/inflammation is the second major upstream event in pathogenesis (see Box 15.1 and Fig. 15.1 ). Thus, an important series of clinical/epidemiological, neuropathological, and experimental studies suggest that maternal intrauterine infection and fetal systemic inflammation are involved in the pathogenesis of a proportion of cases of WMI. Postnatal infections were also significantly associated with increased risk for WMI. The magnitude of the role of infection/inflammation and especially the potential mechanisms thereof are still unresolved issues. Several fundamental questions remain to be resolved. First, rigorous outcome measures to identify and quantify antecedent maternal-fetal infections were frequently lacking. Confirmation of infection by placental pathology was not consistently used in most studies. Second, many studies relied on relatively insensitive neuroimaging modalities to detect WMI, and often, imaging was not obtained in close temporal proximity to the suspected time of infection. Third, although infections were expected to render the white matter more susceptible to injury, there has been little experimental exploration of potential mechanisms of infection-mediated tolerance in preterm white matter. Studies in near-term animals support that the timing of an inflammatory challenge is critical in rendering the brain susceptible or resistant to hypoxia-ischemia. As demonstrated in neonatal rodents, in some settings, a low-grade antecedent infection may protect against more severe injury from a subsequent more severe insult such as hypoxia-ischemia.
Clinical/Epidemiological Observations
Clinical and epidemiological studies suggest a link between maternal and fetal infection, inflammation, and the occurrence of WMI (see Chapters 13 and 16 ). The most commonly held view is that maternal intrauterine infection causes a fetal systemic inflammatory response that results in injury to cerebral white matter. There are several potential mechanisms by which WMI may occur. A fetal systemic inflammatory response may disrupt CBF via cardiovascular insufficiency and secondary cerebral hypoperfusion, as demonstrated in experimental models. As discussed later, inflammation may disrupt cerebral vascular reactivity, as in the setting of chorioamnionitis. For example, inflammation may also potentially trigger direct degeneration of cells in the white matter through mechanisms involving cytotoxic cytokines.
The fetal systemic inflammatory response, recognized for many years, is defined in considerable part by detection of proinflammatory cytokines in blood. A relationship with WMI or associated cerebral palsy in premature infants is suggested by the finding of increased incidence of these outcomes in the presence of (1) evidence for maternal and fetal infection/inflammation (e.g., chorioamnionitis, funisitis, premature rupture of membranes); (2) elevated levels of proinflammatory cytokines, especially interleukin-6 (IL-6) and IL-1β, in amniotic fluid or umbilical cord blood; and (3) evidence for intrauterine T-cell activation. However, nearly all these studies used for the diagnosis of WMI the ultrasonographic finding of echolucencies (i.e., apparent cystic WMI). As noted earlier, cystic WMI now is recognized to account for less than 5% of WMI. Cranial ultrasonography also has poor sensitivity and specificity to detect multifocal noncystic WMI, which comprises the majority of cases (see Chapter 14 ). The potential mis-classification of noncystic WMI as controls makes the interpretation of these data difficult. Moreover, two studies of former preterm infants showed no significant relationship between levels of proinflammatory cytokines in early neonatal blood and the later diagnosis of cerebral palsy.
The role of chorioamnionitis in the pathogenesis of WMI has received considerable attention but remains unresolved because of several important limitations. The majority of early studies used cranial ultrasonography to detect WMI and relied on clinical rather than histopathological criteria. One study of 126 infants showed no correlation between clinical chorioamnionitis and ultrasonographically demonstrated WMI. Some studies used cerebral palsy as an outcome measure rather than assessment of WMI by neuroimaging. Two large meta-analysis studies found a significantly increased risk for cerebral palsy in studies that diagnosed chorioamnionitis by clinical (OR 2.42) or histopathological (OR 1.83) criteria. However, these studies did not evaluate the spectrum of WMI, including more severe cystic WMI, as well as other forms of cerebral pathology that may have contributed to cerebral palsy. Moreover, chorioamnionitis may increase the risk for adverse outcomes related to postnatal infections, necrotizing enterocolitis, or symptomatic hypotension. Greater attention to these postnatal risk factors would strengthen the analysis of the independent contribution of prenatal chorioamnionitis to WMI or cerebral palsy. Few studies have combined MRI-defined WMI with rigorous evaluation of maternal and fetal inflammatory responses by placental pathology to confirm exposure to chorioamnionitis. In a study of 100 consecutive premature infants studied by MRI at term, no significant relationship was noted between the occurrence of chorioamnionitis or prolonged rupture of membranes and moderate to severe WMI. Several additional prospective MRI-based studies similarly failed to find an association between histopathologically confirmed chorioamnionitis and disturbances in brain maturation or injury.
Since as many as 25% of all infants who weigh less than 1500 g at birth experience neonatal sepsis, a role for postnatal infection/inflammation in WMI has been studied. In the aforementioned MRI study of 100 premature infants, a weak relationship was noted between neonatal sepsis and WMI identified at term. Other reports have shown an increased rate of WMI, cerebral palsy, or other neurodevelopmental disabilities in preterm infants after neonatal sepsis. This relationship is particularly apparent among infants of ELBW (401 to 1000 g), of whom 65% exhibit at least one infection during the postnatal period. In a key study of 92 premature infants, postnatal infections and hypotension, but not histologically defined chorioamnionitis, were associated with increased risk for MRI-defined WMI. Despite the lack of strong associations between prenatal chorioamnionitis and adverse neurodevelopmental outcomes, chorioamnionitis may indirectly increase the risk for postnatal infections and hypotension as independent risk factors for cerebral ischemia and WMI.
Potential Role of Proinflammatory Cytokines in White Matter Injury
A potential role for inflammation and, specifically, cytokines in the pathogenesis of WMI has been proposed from human and experimental studies that have detected proinflammatory cytokines in early or advanced stages of WMI. Experimental studies in fetal sheep found that circulating IL1-β and IL-6 cross the blood-brain barrier to access the central nervous system (CNS). Moreover, ischemia-related disruptions in blood-brain barrier permeability were attenuated with neutralizing antibodies directed against either of these cytokines. Perhaps related to these findings are the demonstrations in living premature infants of a relationship between elevated levels of interferon-gamma (IFN-γ) in blood or cerebrospinal fluid (CSF) and ultrasonographically defined WMI or tumor necrosis factor-α (TNF-α) in CSF and MRI-defined WMI. In preterm infants with WMI studied postmortem, IFN-γ was highly expressed in white matter lesions. An additional source of proinflammatory cytokines within the CNS are microglia or astrocytes that are resident in the white matter. In chronic human diffuse WMI, activated microglial are abundantly present.
In experimental models, several microglial-derived inflammatory cytokines have been shown to cause early WMI through peroxynitrite-mediated toxicity to the OL lineage. Bacterial endotoxin exposure similarly may exacerbate WMI via peroxisomal dysfunction and related oxidative stress. Experimental and clinical studies have found that proinflammatory cytokines are detected in association with WMI and can disrupt white matter development in neonatal animal models. The principal cytokines observed in human WMI are TNF-α, IL-1β and IFN-γ. The toxicity of these cytokines to preOLs has been proposed as a potential mechanism for early WMI. Support for this hypothesis is weakened by the lack of several critical observations. Human pathology studies have relied heavily on cytokine staining in chronic WMI samples, but detection of cytokines has not been done in early WMI when the majority of preOL degeneration occurs. Immunohistochemical detection of cytokines in human tissue sections is also a relatively insensitive means to detect differences in cytokine levels. Cytokine detection in human chronic WMI has also been largely restricted to lesions with cystic WMI, whereas the majority of contemporary patients sustain mostly multifocal noncystic WMI. Hence, a role for proinflammatory cytokines in the pathogenesis of the major form of early human WMI remains unresolved.
Delineation of the insult leading to the brain cytokine response in WMI would be of major value in the understanding of pathogenesis . A potential interacting role for systemic infection/inflammation with hypoxia-ischemia has been suggested from experimental studies in which endotoxin exposure was shown to either dampen or potentiate the severity of cerebral injury depending on the timing of exposure. Identification of the initiating insult is difficult in neuropathological studies, but available data suggest a role both for hypoxia-ischemia and systemic infection/inflammation . In the neuropathological series of Kadhim and associates, the 19 cases with WMI and cytokine staining in the lesions had asphyxia in the background, although cases complicated by systemic infection had the greatest staining. Thus, the degree to which hypoxia-ischemia contributed to the inflammatory response in these cases of human WMI is unclear and important to consider. It is well established in animal models of WMI that ischemia/reperfusion is associated with a brisk inflammatory response, characterized by activation of microglial cells, secretion of cytokines, mobilization, adhesion and migration of macrophages and inflammatory cells, and reactive astrocytosis. Persistence of this response, with activated microglia and astrocytes, for many weeks following the insult has been documented in hypoxic-ischemic models in immature animals and in human stroke. As discussed later, activated microglial cells also secrete toxic diffusible products (e.g., reactive oxygen species [ROS] and reactive nitrogen species [RNS]) that may be more injurious than cytokines in the genesis of WMI.
Cellular Mediators of Inflammation
Although the mechanisms by which maternal and fetal infection/inflammation initiate deleterious molecular and structural events in the brain are not fully understood, microglia are present during the early and advanced stages of WMI (see Fig. 15.1 and Chapter 13 ). During normal brain development, microglia have key roles in clearance of apoptotic cells arising from programmed cell death and in synaptic pruning. Microglia are broadly classified in two major phenotypes that are biased toward proinflammatory or antiinflammatory actions. During the immediate early phase of WMI, microglia may initiate or potentiate cell death via ROS and RNS and perhaps glutamate. The mechanisms of ROS/RNS toxicity and excitotoxicity have the potential to contribute to the intrinsic maturation-dependent vulnerability of preOLs to both hypoxia-ischemia and infection/inflammation (see also Chapter 13 ). During the more chronic stages of WMI, the activation state of human microglia has not been defined. It has been proposed that during the progressive remodeling of the white matter after injury, microglia may assume a more antiinflammatory role that could contribute to repair processes to promote oligodendrocyte maturation and myelination in neonatal WMI and multiple sclerosis. This topic is an important direction for future study.
Microglial cells can be identified in normal human brain very early in development, become abundant in forebrain from 16 to 22 weeks of gestation, and are concentrated in cerebral white matter, with a deep-to-superficial gradient . Relatively fewer microglial cells are found in cerebral cortex at this time. In one longitudinal study of human postmortem brain, the density of microglia reached a peak during the period of greatest vulnerability to WMI (third trimester of gestation) and declined markedly in white matter after 37 weeks of gestation. As density declined in cerebral white matter, it increased in cerebral cortex. This observation suggests that a wave of migrating microglia is apparent in cerebral white matter at the optimum time for activation by hypoxia-ischemia or infection or both. Thus, although more data on these developmental features are needed, a maturation-dependent population of cells (i.e., microglia) may be concentrated in cerebral white matter at the right time and location to contribute to WMI when activated .
Infection and Hypoxia-Ischemia-Potentiating Insults
Deleterious interactions between prenatal (maternal or fetal) and postnatal infection/inflammation and hypoxia-ischemia could occur at several steps in the pathway to WMI (see Box 15.1 and Fig. 15.1 ). Thus, such potentiation could develop at the level of the major upstream initiating events of hypoxia-ischemia and infection/inflammation or at the level of the major downstream events of oxidative stress and excitotoxicity. Not unexpectedly, most current evidence supporting a potentiating interaction of infection/inflammation and hypoxia-ischemia emanates from experimental studies (see Chapter 13 ). However, clinical and neuropathological studies of human PVL also support the notion of a potentiating interaction.
Inflammation-Induced Sensitization of White Matter Injury
Both experimental and clinical studies provide support for potential interactions between distinct injurious factors that may potentiate or reduce the severity of WMI. Although most of the experimental studies have focused on sensitization of the term infant brain, they provide an important new direction to define the impact of multifactorial events on the progression of WMI during the early phase of cell death and the later stages of regeneration and repair. Of particular importance to the pathogenesis of early WMI is the notion that infection/inflammation may potentiate or mitigate WMI arising from cerebral hypoxia-ischemia .
As discussed earlier, antenatal or postnatal infection/sepsis may have adverse systemic and cerebral circulatory effects that contribute to WMI. Major disturbances in the regulation of CBF and cerebral oxygen delivery have been observed in fetal sheep after relatively low doses of lipopolysaccharide. Significant episodes of chorioamnionitis are associated with funisitis, fetal and postnatal hemodynamic instability, and signs of persistent inflammatory states. For example, in one study of 61 consecutively born preterm infants (<32 weeks of gestation), the risk of WMI, identified by MRI and ultrasonography, was enhanced with a history of histological chorioamnionitis only in the presence of concurrent placental vascular disturbances consistent with a placental perfusion defect. Whether the latter was caused by intrauterine infection was not clear, although such a relationship has been shown. Histological chorioamnionitis without placental perfusion defect was not associated with white matter disease.
Because several cytokines stimulated by infection, especially TNF-α and IL-1β, exhibit vasoactive properties, it is reasonable to postulate that these inflammatory molecules could contribute to impaired cerebrovascular autoregulation, likely important in the pathogenesis of WMI (see earlier discussion). Hypoxia-ischemia ( without infection ) also leads to both systemic and cerebral elevations of vasoactive proinflammatory cytokines (e.g., TNF-α and IL-1β) derived from peripheral monocytes and brain microglia. A resident population of microglia is concentrated in human preterm cerebral white matter with the potential to be activated and become injurious during the early phase of WMI (see earlier). Thus, activated microgliosis is a consistent and prominent feature of early and chronic white matter lesions with the potential to exert vasoactive effects that may promote or amplify the extent of WMI due to hypoxia-ischemia.
It is also important to consider the potential impact of subclinical factors that in isolation would not be injurious but in combination may synergize to potentiate WMI. The combination of infection/inflammation and hypoxia-ischemia could result in deleterious systemic circulatory effects that ultimately affect the cerebral circulation (see Fig. 15.1 ). Many other maternal or iatrogenic factors may also induce fetal stress and alter the susceptibility to hypoxia-ischemia.
Although in some experimental studies, deleterious systemic hemodynamic effects have been shown to accompany fetal or neonatal infection or exposure to lipopolysaccharide or cytokines, equally important to consider is the concept of inflammation/infection mediated tolerance to a hypoxic-ischemic event, which may reduce the severity of WMI . Mechanisms of ischemic tolerance/preconditioning have been extensively described in neonatal hypoxia-ischemia. Studies in neonatal rodents have demonstrated that the timing of an inflammatory challenge relative to subsequent hypoxia-ischemia defines the severity of cerebral injury, which may be attenuated or enhanced .
Thus, taken together, a growing body of experimental, neuropathological, and clinical evidence suggests that infection/inflammation and hypoxia-ischemia may interact to influence the magnitude of WMI . The identification of mechanisms of sensitization would have major implications concerning the means of surveillance of infants at risk and the approaches to prevention and are critical for future research.
Intrinsic Vulnerability of the Premature Newborn to Acute Cerebral White Matter Injury
The cerebral white matter of the premature infant is intrinsically susceptible to a broad spectrum of injury (see Box 15.1 ). At the more severe end are the now relatively rare severe destructive lesions that result in microscopic or macroscopic cystic white matter necrosis and the nonselective degeneration of all cell types within these lesions. In addition to glia, migrating and interstitial white matter neurons, subplate neurons, and axons may degenerate in necrotic lesions, as supported by both human and experimental studies. Neuroimaging studies support that the majority of infants sustain focal or diffuse non-necrotic white matter lesions. Neuropathology studies support that these nondestructive lesions involve a maturation-dependent intrinsic vulnerability of the cerebral white matter that targets the oligodendrocyte lineage. This intrinsic vulnerability is supported by experimental studies (see Chapter 13 ), by the decline in incidence of relatively restricted WMI later in gestation, by the concentration of vulnerable preOLs during the peak time period of occurrence of the lesion, and by the selective degeneration of preOLs in the early lesions. The intrinsic vulnerability relates principally to the two major downstream mechanisms of WMI (i.e., free radical attack by ROS and RNS and excitotoxicity; see Box 15.1 ). The details of the intrinsic vulnerabilities, as derived from experimental studies, are described in Chapter 13 . Here are emphasized studies performed on human premature brain that have defined a role for preOL death as a key initiating event in the pathogenesis of chronic WMI as discussed later.
Vulnerability to Free Radical Attack
Both experimental and human pathology studies support that preOLs selectively degenerate during the early phase of WMI. A role for oxidative stress in preOL degeneration is supported by in vitro, experimental, and human neuropathology studies ( Box 15.4 ). The fetal cerebral white matter is rich in membrane lipids that are readily oxidized. The developing white matter may be more vulnerable to oxidative damage due to a delay in the expression of the antioxidant enzymes, superoxide dismutases-1 and -2, catalase, and glutathione peroxidase, but expression data in rat and human have not been confirmed with enzyme activity data. Although controversy exists regarding the role of RNS in the pathogenesis of early WMI, human preterm white matter is especially prone to lipid peroxidation-mediated injury. Both the biochemical and immunohistochemical detection of protein, lipid, and DNA adducts generated by reaction oxygen and nitrogen species (e.g., protein carbonyls, 4-hydroxynonenal and 8-oxo-deoxyguanosine) are unreliable in human preterm WMI. Sensitive detection of the aldehydes formed from lipid hydroperoxides is specifically achieved in glia by measurement of F 2 -isoprostanes, which are stable adducts in postmortem human tissue. F 2 -isoprostanes were significantly elevated in histopathologically confirmed cases of early WMI and coincided with the levels detected in the cerebral cortex where severe perinatal asphyxia was confirmed. This oxidative damage was accompanied by a pronounced increase in degenerating cells in the periventricular white matter, which were shown to be preOLs ( Fig. 15.9 ). F 4 -neuroprostanes, a specific marker of oxidative injury to neuronal elements, were not detected in white matter or cerebral cortex, consistent with the notion that neurons and axons were spared ( Fig. 15.10 ). Hence, oxidative damage selectively targeted the preterm white matter, and the degree of oxidative damage sustained in the early phases of WMI is considerable and comparable to that sustained in severe perinatal asphyxia from hypoxic-ischemia.
- ◆
Detectable levels of staining for the iron binding protein ferritin in preterm human white matter suggest the possibility of iron-mediated generation of reactive oxygen species during hypoxia-ischemia.
- ◆
Reduced staining for antioxidant enzymes (superoxide dismutase1 and 2, catalase, and glutathione peroxidase) in preterm human white matter suggests reduced antioxidant defenses.
- ◆
Preterm human early WMI displays elevated levels of markers of lipid peroxidation by staining and by biochemical detection of F 2 -isoprostanes.
- ◆
Preterm human cerebral cortex and adjacent regions of early WMI display low levels of F4-neuroprostanes, a marker of oxidative damage to neurons and axons.
WMI, White matter injury.


The mechanisms underlying the maturation-dependent vulnerability of developing white matter to ROS attack has been extensively studied in an attempt to design protective strategies against WMI (see also Chapter 13 ). Definition of the timing of ROS generation requires animal studies. Studies in a mid-gestation fetal sheep asphyxial model of preterm WMI found that the peak of free radical generation was delayed and not detected until several hours after the insult. This timing likely reflected a delayed wave of ROS generation that occurs during the peak of cell death. Studies in a preterm fetal rabbit model of placental insufficiency detected increased superoxide production during the immediate ischemia-reperfusion period. Early delivery of a superoxide dismutase mimic or antioxidants significantly reduced hypertonia in this model of mixed cerebral palsy. Early superoxide generation is consistent with the delay in expression of copper-zinc superoxide dismutase, manganese superoxide dismutase, and catalase in preterm human white matter. The possibility that superoxide generation promotes the accumulation of hydrogen peroxide and its conversion to the hydroxyl radical by the Fenton reaction is suggested by the early appearance of iron in developing human white matter, as well as by the acquisition of iron by developing oligodendrocytes for differentiation. Supportive of a relationship between iron and WMI is the observation that, for many weeks after human intraventricular hemorrhage, a disorder that sharply increases the risk of WMI, CSF levels of non–protein-bound iron are markedly increased. In addition, several reports suggest that the vulnerability to oxidative attack in the premature white matter shares similarities with systemic features. Studies of plasma of human premature infants indicated a propensity to generate free radicals, including the hydroxyl radical; increases in plasma non–protein-bound iron, accentuated by hypoxia or blood transfusion; and impaired antioxidant defenses. Taken together, the findings support a maturation-dependent window of vulnerability to oxidative attack during the period in preterm white matter development when preOLs predominate in the white matter (see Chapter 13 and Box 15.1 ).
Vulnerability to Excitotoxicity
As detailed in Chapter 13 , glutamate is capable of inducing maturation-dependent death of preOLs by non–receptor-mediated and receptor-mediated mechanisms. The non–receptor-mediated mechanism involves glutamate competition for the cystine transporter and promotion of cystine efflux under conditions of high extracellular levels of glutamate. Depletion of intracellular glutathione (which requires cysteine for biosynthesis) is accompanied by cell death by oxidative stress. However, the substantial levels (millimolar) of glutamate required for this effect suggest that this mechanism may not operate in vivo under most pathological conditions. By contrast, the receptor-mediated mechanism , which requires micromolar levels of glutamate, is more likely to occur in vivo, as shown directly in animal models (see Chapter 13 ). Both α-amino-3-hydroxy-5-methyl-4-isoxazolepropionic acid (AMPA) and N -methyl- d -aspartate (NMDA) receptors appear to be involved.
The principal sources of elevated extracellular glutamate in cerebral white matter with hypoxia-ischemia are glutamate transporters (see Chapter 13 ). Glutamate transporters constitute a family of 5 human genes, EAAT1-5. The important transporters in the forebrain are EAAT1-3. All three transporters are detected in developing white matter, but expression in preOLs has not been specifically defined.
Failure of glutamate uptake and actual reversal of transport occur in the setting of energy failure because of the failure of the high-affinity sodium-potassium ion pump. Experimental in vitro studies support the concept of a fatal feedback loop in developing OLs whereby in the setting of energy failure, reverse glutamate transport occurs to provide a source of extracellular glutamate. When activated, glutamate receptors expressed on the same cells mediate excessive influx of calcium, which triggers excitotoxic injury and cell death. This model of excitotoxic injury in the immature brain contrasts with that in the mature brain and may be a cell-autonomous process in which certain populations of cells provide both the source and the target for pathological accumulations of glutamate.
Excessive glutamate receptor activation during ischemia requires an elevation in extracellular glutamate and a concomitant glutamate loss from at least one cellular compartment. The potential sources of glutamate release to cerebral white matter include astrocytes, OLs, axons and cells of the choroid plexus. In experimental perinatal hypoxia-ischemia studies, depletion of glutamate in vivo was most pronounced in axons and premyelinating stages of the OL lineage, which suggested that re-uptake mechanisms may be immature in the perinatal brain or dysfunctional during hypoxia-ischemia.
The critical initiators of excitotoxic cell death are glutamate receptors . Focal expression of NMDA receptors on the processes of OL progenitors and mature OLs in vivo mediates a rapid, Ca 2+ -dependent, disintegration of OL processes under in vitro conditions that mimic ischemia, and NMDA receptors have been found on premyelinating oligodendrocytes in developing human white matter. As described in Chapter 13 , glutamate toxicity to preOLs in vitro is also mediated by ionotropic glutamate receptors (iGluRs) of the AMPA/kainate type. Although these in vitro studies suggest that calcium-permeable AMPA receptors on preOLs might be important in WMI, there remains uncertainty about whether preOLs in vivo express this type of receptor. Cells deficient in the GluR2 subunit render them Ca 2+ permeable and thereby capable of toxicity. Increased expression of the GluR4 subunit occurs between 23 and 32 weeks of gestation in human parietal white matter and P7 rat corpus callosum. The timing of calcium permeable AMPA type glutamate receptor expression and NMDA receptor expression during human and rat white matter development thus appears to coincide with the window of heightened susceptibility to WMI, but there remains uncertainty about whether human preOLs in vivo express these types of receptors during the peak period for WMI during preterm white matter development. Further in vivo studies are needed to verify the direct toxicity of glutamate to preOLs, other OL lineage stages, and astrocytes. NMDA and AMPA/kainate receptor antagonists prevented myelin loss after perinatal hypoxia-ischemia in the term equivalent rat. However, the efficacy of glutamate receptor antagonists to directly block degeneration of preOLs and axons in vivo remains largely unstudied.
Other Potentially Vulnerable White Matter–Associated Cellular Elements
Preterm cerebral white matter contains numerous neurons that include transient populations (e.g., migrating neuronal progenitors and subplate neurons) as well as interstitial neurons that appear to reside permanently in the white matter. Axons are in a state of very active development in cerebral white matter of the human premature infant. The confluence of developing ascending and descending projection fibers, commissural fibers, and sagittal corticocortical association fibers in the peritrigonal region is particularly prominent at this time and may also degenerate in more severe WMI.
Data from experimental studies found that immature neurons in preterm white and gray matter are surprisingly resistant to moderately severe hypoxia-ischemia that is of sufficient magnitude to cause diffuse WMI and selective preOL degeneration. Not unexpectedly, both neurons and axons nonselectively undergo necrotic degeneration in more severe destructive lesions that diffusely target glia as well. Subplate neurons were initially proposed to be selectively vulnerable to hypoxia-ischemia in neonatal rats, but subsequent extensive studies found that this transient population degenerates primarily in severe lesions in association with other populations of cortical neurons. In more severe WMI, preterm human subplate neurons degenerate in lesions where other populations of neurons also degenerate. In chronic diffuse noncystic WMI, the subplate and adjacent regions display evidence of sustained inflammatory responses. Studies in preterm fetal sheep found that diffuse WMI was not accompanied by acute or delayed degeneration of migrating neurons destined to become GABAergic interneurons. A single small neuropathological study found a decrease in GABAergic neurons in the white matter of infants with severe necrotic WMI, but other classes of neurons that typically degenerate in such lesions were not studied. More data are needed on this issue.
Axonal degeneration is a prominent feature of WMI when necrosis is identified. For example, axonal injury has been observed in regions of chronic human diffuse WMI that are adjacent to regions of necrosis, but these dystrophic axons are likely to be structurally continuous with the degenerating axons in necrotic foci. Necrotic lesions are a minor component of WMI in both recent human studies and experimental models and comprise only about 5% of the total burden of WMI. During the acute phase of diffuse WMI in preterm fetal sheep, axonal injury was rarely observed, and in chronic diffuse WMI, no significant axonal degeneration, axonal loss, or shift in the distribution of axon calibers was observed by quantitative electron microscopy studies. Hence, the major sites of axonal degeneration are necrotic lesions, and axons appear to be structurally intact in diffuse WMI. The functional integrity of axons in diffuse lesions is difficult to study and is an important direction for future study.
Factors Related to the Progression of Chronic White Matter Injury
As discussed earlier, focal necrotic WMI results in myelination failure, which is likely to be permanent, because it is typically accompanied by loss of all glial cells, including preOLs and astrocytes, as well as loss of axons that are required for myelination ( Fig. 15.11 ). The pathogenesis of focal necrotic WMI is distinct from the processes that lead to non-necrotic diffuse WMI ( Box 15.5 ). The evolution of diffuse WMI comprises two major overlapping events that contribute to a process of disrupted regeneration and repair of chronic lesions with disturbances in the normal progression of oligodendrocyte lineage maturation and myelination (see Fig. 15.11 ). The first event involves an early cell proliferative response to WMI in which early oligodendrocyte progenitor cells increase in subacute lesions. This plasticity response results in regeneration of preOLs that have degenerated during acute WMI. The second overlapping event occurs over days to weeks and involves a disruption in the normal maturational processes by which preOLs differentiate to myelinating oligodendrocytes. Hence, despite the pronounced selective degeneration of preOLs in early diffuse WMI, disturbances in myelination in chronic lesions do not appear to arise from a loss of myelinating cells but rather from newly generated oligodendrocyte progenitor cells (OPCs) that fail to fully mature (see earlier).
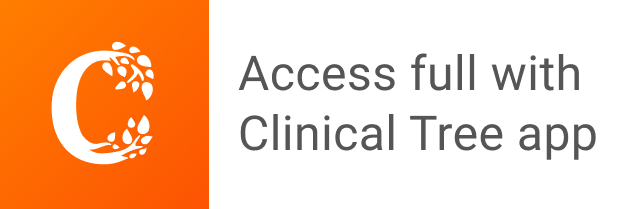