Fig. 10.1
Mechanisms regulating cellular changes in the two neurogenic compartments following traumatic brain injury (TBI). Similarities and differences exist between TBI-induced responses in the SVZ (A-D) and dentate gyrus (E-H). a Under naïve conditions in the SVZ-RMS NSPCs (purple) line the lateral ventricles and produce neuroblasts (orange/green) that migrate through the RMS to the olfactory bulb. b Following TBI, NSPCs are stimulated to proliferate and survive post-injury.Expression of multiple growth promoting molecules are enhanced and contribute to the proliferative effects. Ephrins and Eph receptors, negative regulators, are down regulated during population expansion. Low oxygen levels (blue) precede proliferative changes in the SVZ-RMS and may activate key pathways necessary for proliferation and migration effects. c NSPCs and neuroblasts actively migrate to the peri-lesion site after TBI. While the mechanism(s) regulating this event are under investigation, the SDF/CXCR4 has been implicated. d Differentiation of SVZ-derived cells occurs in the damaged cortex. Several key factors that regulate gliogenesis in these cells also promote oligodendrogenesis. The survival and integration of newborn neurons remains limited under these conditions. e Neurogenesis in the dentate gyrus. f Unlike the SVZ, neuroblasts and immature neurons are depleted due to necrosis and apoptosis, the main inducer of which is still unknown. g Proliferation of NSPCs counterbalances immature neuronal loss in the DG and is positively regulated by several known factors. h Restoration of mature granule neuron numbers is mediated by NSPC expansion and can be enhanced by delivery of growth factors. SVZ subventricular zone, RMS rostral migratory stream, ML molecular layer, GCL granule cell layer, SGZ sub-granule zone, EGF epidermal growth factor, FGF fibroblast growth factor, DEtA/NONOate nitric oxide donor, VEGF vascular endothelial growth factor, BDNF brain-derived growth factor, NGF nerve growth factor, Epo erythropoietin, SDF stromal-derived growth factor, GCSF granulocyte colony stimulating factor, Thbs4 thrombospondin-4, NMDA N-Methyl-D-aspartic acid
10.2 Effects of TBI on the Neurogenic Compartments
The number of NSPCs in both the SVZ and hippocampus are significantly increased after TBI, a process that is more robust in juvenile animals (Singer et al. 2011; Kleindienst et al. 2013; Taylor et al. 2013). NSPCs from the SVZ appear to have the potential to migrate to areas of focal cortical damage, while hippocampal neurogenesis occurs in the setting of diffuse injury. Overall, the number of new neurons generated after TBI remains small in comparison with astrocyte and oligodendroctye differentiation . The following sections will examine injury-induced responses in these two prominent neurogenic compartments in the adult brain.
10.2.1 Neurogenesis in the SVZ
The SVZ represents the remnant of an enlarged perinatal ventricular germinal zone which narrows during development and persists throughout adulthood as a neurogenic compartment consisting of multiple cell lineages that derive from an original self-renewing stem cell . (Tramontin et al. 2003). This relatively quiescent or slowly proliferating cell expresses glial fibrillary acidic protein (GFAP; type B cells) and differentiates to become a rapidly dividing transit-amplifying progenitor (type C cells) which generate the typically seen chain and migrating neuroblasts in the rostral migratory stream (RMS) (type A cells) (Alvarez-Buylla and Garcia-Verdugo 2002). Interestingly, during development the SVZ generates both neuronal and glial cell types of the CNS; however, only neurogenesis (birth of new neurons) persists in adulthood. The normal role for adult NSPCs in the anterior telencephalic SVZ is to supply the RMS with neuronal precursors that continually replace glomerular neurons in the olfactory bulb (Luskin 1994; Pencea et al. 2001). Continuous production of neuroblasts that migrate and integrate into the olfactory bulb circuitry is controlled by the local environment within the SVZ niche, which is separated from the ventricle cavity by ependymal cells (Lois and Alvarez-Buylla 1993). It is generally thought that enhancing the neurogenic activities of endogenous NSPCs may provide additive therapeutic benefits after brain injury.
Consistent with models of ischemia , experimental TBI induces an increase in SVZ proliferation, and a secondary redirected stream of precursors into the surrounding tissues (Salman et al. 2004). Several rodent models of TBI have been extensively used to demonstrate increases in bilateral proliferation in the adult SVZ up to 14 days after injury; namely the lateral fluid percussion (LFP) injury (Chirumamilla et al. 2002; Yoshimura et al. 2003; Urrea et al. 2007; Bye et al. 2011) and unilateral controlled cortical impact (CCI) models (Chirumamilla et al. 2002; Lu et al. 2003; Ramaswamy et al. 2005; Urrea et al. 2007; Theus et al. 2010; Radomski et al. 2013). This effect has not been tested in blast-induced or repetitive TBI injury models to date. These proliferative changes have been shown to persist at least one year following LFP injury in rats and is linked to progressive ventriculomegaly (Chen et al. 2003), whether these cells continue to migrate to areas of active neural repair at this time has yet to be determined. TBI-induced proliferation in the SVZ also appears to correlate with injury severity. Recent studies show that both mild and moderate TBI alters the SVZ-RMS niche with a greater proliferative response seen following moderate CCI injury. Interestingly, this translates into defects associated with ipsilateral but not contralateral olfactory bulb neurogenesis and impaired olfaction up to 30 days post-injury (Radomski et al. 2013). TBI-induced proliferation in the SVZ niche does not result in a corresponding increase in olfactory bulb neurogenesis. This is most likely due to the mass exodus of cells migrating from the SVZ-RMS system to the lesion and peri-lesion sites which counterbalances the increases in proliferation. Regional changes in numerous soluble neurotrophic factors such as nerve growth factor (NGF), brain-derived trophic factor (BDNF) and fibroblast growth factor-2 (FGF-2) have been implicated in stimulating proliferation in the SVZ following TBI (Oyesiku et al. 1999; Truettner et al. 1999; Yoshimura et al. 2003) (see Table 10.1). The differential interactions among these and other neurotrophins may be important in determining the final number and phenotype of the SVZ-responding cells. The morphogens Wnt, sonic hedgehog (Shh) and bone morphogenic proteins (BMPs) may also play a major role in the NSPC response to brain injury. For example, Wnts are endogenously expressed in the adult SVZ (Wexler et al. 2009) and β-catenin activity has been shown to be up regulated in proliferating progenitor cells after TBI (White et al. 2010). Whether these and other diffusible molecules, seen to be enhanced in the SVZ after TBI, are directly related to the expansion and migration effect has yet to be determined.
Table 10.1
Positive and negative regulators of adult neurogenesis in the traumatic injured brain
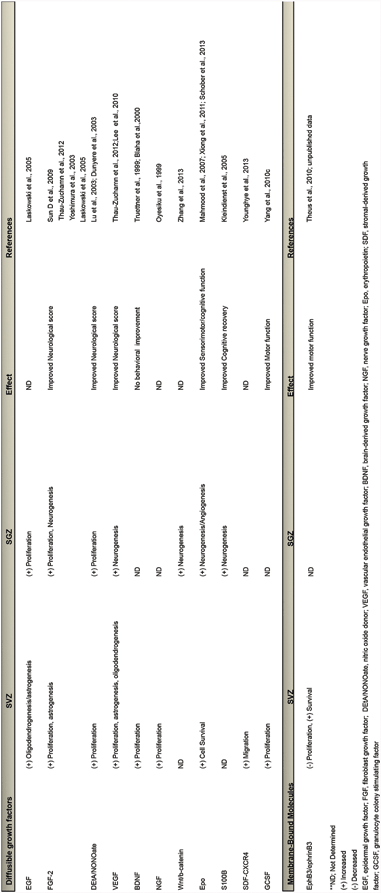
In addition to soluble mitogenic factors that may participate in stimulating the SVZ response to TBI, studies have demonstrated changes in the EphB3 receptor, a local inhibitory molecule that maintains homeostasis in the SVZ (Theus et al. 2010). In contrast to soluble mediators of NSPC functions, ephrins and Eph receptors are membrane-bound signaling partners of both A and B-classes that have recently been shown to tightly regulate proliferation and migration in the SVZ (Conover et al. 2000; Holmberg et al. 2005; Furne et al. 2009). The mechanisms controlling their expression in this region are not fully understood and have not been, until recently, studied in the context of brain injury. Examination of the temporal change in EphB3 expression showed a dramatic decrease which correlated with CCI-induced proliferation, cell survival and lowered oxygen conditions or hypoxia in the SVZ (Theus et al. 2010; Baumann et al. 2013). In addition to TBI-induced proliferation, cell death or turnover is reduced in the SVZ, an underappreciated and under studied cellular response in the neurogenic compartment. These studies further identified a novel mechanism controlling cell survival of the NSPCs involving EphB3 and EphA4 as dependence receptors in a cell autonomous fashion (Furne et al. 2009; Theus et al. 2010; Nelersa et al. 2012). Overall, the removal of such inhibitory control on adult neurogenesis may be an early event that allows simultaneous expansion and migration of the NSPC population in response to TBI-induced neurotrophic signals produced after trauma.
Migration of SVZ-derived neural stem and/or progenitor cells, analyzed using lentiviral, microsphere and dye labeling, has been demonstrated to occur following TBI (Salman et al. 2004; Ramaswamy et al. 2005; Saha et al. 2013). Although, the mechanism(s) regulating their recruitment are unknown, these cells migrate to the striatum, corpus callosum (CC), and peri-lesion cortex, but not the hippocampus , and differentiate mainly in glial cells, which may contribute to glial scar formation. Many of these migrating cells are positive for doublecortin (DCX), a marker of immature neuroblasts, suggesting that local neurogenesis could be driven by injury-specific extracellular cues. In the peri-lesion cortex, expression of the mature neuronal marker NeuN was seen in cells tagged prior to injury by intraventricular infusion of a lipophilic dye, suggesting that these cells were derived from the SVZ compartment. Whether neuroblasts that migrate from the SVZ can effectively replace cortical neuronal loss caused by TBI remains to be determined. Similarly, it is conceivable that migrating NSPCs could be directed toward an oligodendrocyte lineage to aid in remyelination of white matter tracks damaged by TBI. The potential for directing the phenotypic differentiation of SVZ-derived cells, that have been clearly shown to migrate to areas of injury, may become an intriguing therapeutic goal. On the other hand, the SVZ has been shown to be extremely heterogenic and the fact that progenitor populations existing in different subregions (Doetsch et al. 1997; Sundholm-Peters et al. 2004; Azim et al. 2012) may respond differently to trauma is underappreciated. Therefore, a better understanding of the cellular and molecular cues driving the directed migration and differentiation of SVZ-derived cells to areas of tissue damage is needed in the context of TBI.
Significant spontaneous functional recovery has been shown to occur following TBI (Anderson et al. 2000; Demeurisse 2000; Sinha et al. 2013); injury-induced neurogenesis has been thought to be one potential contributor to this effect (Chirumamilla et al. 2002; Chen et al. 2003; Richardson et al. 2007). Several studies have shown that a variety of pharmacologic agents increase neurogenesis and lead to improved outcomes following TBI. The drugs, however, have effects on the brain that are independent of their ability to promote neurogenesis, and it therefore becomes difficult to attribute improvements in behavior to their effects on the neurogenic compartments. Although it is well-established that SVZ progenitors are activated by injury, the relevance of this response and the mechanism(s) responsible still remain elusive. In order to demonstrate that functional recovery requires NSPC activation, more cell-specific assays are needed. Several experimental approaches have been used to test the contribution of NSPCs to spontaneous recovery after CNS injury. Specifically, the generation of genetically engineered mice that can regulate neurogenesis more directly, using an inducible system to drive the expression of the herpes simplex virus thymidine kinase (HSV-TK), have aided in testing the functional significance of NSPCs by ablating them in a temporally controlled manner (Singer et al. 2009; Sun et al. 2013). Other methods such as administration of Ara-C or cranial irradiation have also been used; however, lack of specificity and potentially toxic side effects that lead to unwanted immune activation may affect other mediators of recovery or damage not related to neurogenesis (Doetsch et al. 1999; Naylor et al. 2008; Hellstrom et al. 2009; Lau et al. 2009).
Using a genetic approach, studies have demonstrated a supportive role for NSPCs in the stabilization of the cortical microenvironment after TBI. Administration of ganciclovir (GCV) was used to ablate actively dividing NSPCs expressing HSV-TK under the control of the nestinδ promoter, which drives expression selectively in neural progenitors (Yu et al. 2008). Ablation of the NSPC population in the adult brain attenuated spontaneous functional recovery, exacerbated neuronal injury, promoted astrocyte hypertrophy and reduced the local glial response (unpublished findings). These findings indicate that NSPCs play a role in TBI-induced astrogenesis by contributing to glial numbers and by limiting local resident glial reactivity to support recovery of the injured tissue. This is consistent with previous reports showing that SVZ-derived astrocytes, which have different properties compared with cortical astrocytes namely high levels of thrombospondin 4 (Thbs4), represented the majority of migrating SVZ-derived NSPCs that target the acute injured cortex and not doublecortin (DCX + ) neuroblasts at 2 weeks post-photothrombotic injury (Benner et al. 2013). Interestingly, Thbs4 knockout mice show a phenotypic switch of the fate labeled SVZ-derived cells present at the injury site, where DCX + cells predominated over GFAP expressing cells. Thbs4 regulates this response by directly activating Notch1 receptor. Notch signaling in neural stem cells strongly promotes gliogenesis and reduces neuronal fate. This altered cellular response resulted in abnormal glial scar formation and increased microvascular hemorrhage . It is unclear, however, whether the DCX + migrating neuroblasts survived and fully differentiated into appropriate functionally mature neurons in the damaged cortex during sub-acute and chronic phases of injury. Eventual neuronal replacement from these cells might not be successful without first stabilizing the injured environment.
It is evident from these studies that the migrating SVZ-derived NSPCs do indeed play an important role in the acute response to TBI and other types of brain injuries through astrogliosis and trophic support. It also supports the idea that highly migratory SVZ-derived NSPCs are amenable to phenotypic switching , although the potential of these cells to generate oligodendrocytes following TBI needs to be determined. During post-natal development, NSPCs generate oligodendrocytes that integrate into white matter (Levison and Goldman, 1993) which ceases in adulthood. However, reactivation following demyelinating conditions such as EAE or lysolecithin-induced demyelination has been shown to mobilize the cells to the CC and striatum where they differentiated into astrocytes and oligodendrocytes (Picard-Riera et al. 2002a; Nait-Oumesmar et al. 2008). Recent studies indicate that a subpopulation of epidermal-growth-factor (EGF)-activated type-B cells are oligodendrocyte precursor cells (OPCs) and contribute to myelin repair in the CC following a demyelinating lesion and traumatic axonal injury (Gonzalez-Perez and Alvarez-Buylla 2011; Sullivan et al. 2013). Therefore, different subpopulations of SVZ-derived cells (i.e. neural stem, transit-amplifying, OPC progenitor and neuroblast) may contribute to injury stability and repair by migrating to multiple regions of damage following trauma, a mechanism(s) that depends on the local microenvironmental cues. The potential for directing the phenotypic differentiation of endogenous NSPCs may become an intriguing therapeutic goal, once the molecular signals driving these phenomena are better understood.
To determine whether enhancing neurogenesis can improve functional outcomes following TBI, studies have examined several growth factors that regulate the expansion and differentiation of SVZ-derived cells in injured tissues. In particular, pharmacologic studies using exogenously administered growth factors have correlated increases in proliferation , survival and migration of SVZ-derived cells with greater functional recovery after TBI (Sun et al. 2009, 2010; Thau-Zuchman et al. 2010; Yang et al. 2010). Among these factors, basic fibroblast growth factor (bFGF), epidermal growth factor (EGF), platelet-derived growth factor (PDGF), BDNF, NGF, erythropoietin (Epo) and vascular endothelial growth factor (VEGF) have been widely accepted as important mediators of neurogenesis and potent mitogenic factors for NSPCs in both in vitro and in vivo settings (Alagappan et al. 2009, 2013; Mani et al. 2010; Alvarez-Palazuelos et al. 2011; Bath et al. 2012; Moore et al. 2013; Woodbury and Ikezu 2013). For instance, peripheral administration of Epo promoted NSPC proliferation, neurogenesis, oligodendrogenesis, and neurovascular remodeling following TBI in rats that led to enhanced spatial learning performance and sensory motor function (Ning et al. 2011; Xiong et al. 2011). These studies suggest that growth factor treatment could augment NSPC activity directly resulting in identifiable improvements in functional recovery. A number of studies show that diffusible trophic factors may be suitable for expanding the population of NSPCs in the early phase of repair following TBI, while others have been shown them to direct cell fate choice on select NSPC sub-populations (Alvarez-Palazuelos et al. 2011). Based on these observations, strategies to expand the entire neurogenic compartment prophylactically or immediately following injury would be ideal in order to provide greater numbers of NSPCs that are able to migrate to areas of injury and stabilize the microenvironment. Once stabilized, such treatments may be modified with delivery of additional factors better able to control neuronal or oligodendrocyte cell fate determination. Although, neuronal replacement strategies using this endogenous repair mechanism could further enhance recovery, it has yet to be determined whether these olfactory-bound NSPCs are capable of differentiating into excitatory neurons with appropriate cortical projections in traumatic injured tissues. Likewise, it is unclear whether providing activity-dependent dendritic input is necessary for long-term survival of newly generated cortical neurons.
10.2.2 Neurogenesis in the Hippocampus
While neurogenesis in the SVZ contributes to olfactory function, the generation of new neurons within the DG of the adult hippocampus is critical for spatial learning, object recognition and memory . Although selectively more vulnerable to TBI, the neurogenic region of the hippocampus may have a unique ability to locally replace damaged neurons, as this injury site is normally an area of active endogenous neurogenesis . Studies in both rodents and humans demonstrate that cells with astrocyte morphology reside in the SGZ of the DG and continually generate neuroblasts, which migrate into the granule cell layer (GCL), differentiate into mature excitatory neurons and extend axons into the CA3 region (Eriksson et al. 1998; Roy et al. 2000; Seri et al. 2001), whereas SVZ neuroblasts mainly contribute to the inhibitory interneuron population in the OB. Although some of these new neurons die by apoptosis over a few weeks, many demonstrate functional integration and evoked electrical potential within the hippocampal circuitry (Hastings and Gould 1999; Markakis and Gage 1999; van Praag et al. 2002). Unlike the SVZ, SGZ-derived NSPCs are relatively quiescent and have a much slower proliferative rate (Seri et al. 2001) and subsequent neuroblast production undergo short-distance migration into the GCL. Long distance migration does not seem to occur in pathological situations like it does in the SVZ (Picard-Riera et al. 2004; Brus et al. 2013). Additionally, temporal profiling of these cells indicate that differentially regulated, cell-autonomous factors change over time which could make these cells more or less responsive to niche cues during normal aging (Gilley et al. 2011). Many studies have highlighted the presence of increased neurogenesis in the injured DG. These studies, performed in multiple models of injury and disease, demonstrate that new SGZ-derived neurons are able to replace lost neurons resulting in restored function of the hippocampus (Nakatomi et al. 2002; Jin et al. 2003; Lu et al. 2003; Naylor et al. 2008; Ning et al. 2011) .
One of the functional hallmarks of TBI is the pronounced deficit in learning and memory, which is usually associated with the histological presence of cell death in the DG, CA1 and CA3 layers (Smith et al. 1991, 1994, 1995; Hamm et al. 1993; Reeves et al. 1997; Rola et al. 2006). Because increased neurogenesis in this region has been linked to enhanced learning and memory function, injury-induced neurogenesis could function to replace damaged neurons, and thus contribute to neuronal circuit repair and restoration of neurological function in patients with TBI. Determining how this normally latent pool of NSPCs can be activated or even enhanced offers considerable potential for the development of targeted neurogenic-based therapeutics to ameliorate acute and chronic TBI-induced cognitive decline . Recent BrdU-labeling and cell lineage tracing studies using transgenic mice carrying an inducible yellow fluorescent protein (YFP) gene under the nestinδ promoter, indicate that TBI evokes a sustained increase in neurogenesis in the adult hippocampus lasting up to 6 months post-TBI and exerts differential effects acutely in hippocampal DG-derived progenitors depending on their state of lineage progression (Kernie et al. 2001; Yu et al. 2008; Singer et al. 2009). For example, through unknown mechanisms the early, nestin-expressing progenitors are stimulated to divide after CCI and LFP injuries (Rice et al. 2003; Sun et al. 2005; Yu et al. 2008), whereas the late DCX-expressing neuroblasts or immature neurons are extremely vulnerable to brain injury and undergo acute selective cell death (Yu et al. 2008; Zhou et al. 2012). Cell death is observed in the ipsilateral SGZ, GCL and occasionally in the hilus following CCI-injury. Cell death begins as early as 6 h, peaks at 24 h and reaches normal levels at 14 days post-injury (Rola et al. 2006). It appears that the time course of cellular loss of GCL neurons and SGZ neuroblasts in the DG mimics that of the cortical injured site. Conversely, few dying cells are seen in the contralateral DG. Surprisingly, while the late neuroblast progenitors are selectively vulnerable to cell death in the SGZ, cell survival is actually enhanced in the SVZ (Theus et al. 2010). The differences in their vulnerability may be related to the proximity of the neurogenic compartment to the injury, specific niche cues or intrinsic factor expression regulating cell autonomous activities .
It is currently unknown why immature neurons in the DG are so vulnerable to TBI. Recent studies of newborn neurons in the dentate gyrus show that they are electrophysiologically distinct from mature granular neurons in the dentate gyrus (Tovar and Westbrook 1999; Lopez de Armentia and Sah 2003; Ye et al. 2005; Ge et al. 2006). Newborn immature neurons are also more easily excited than mature neurons due to their unique ion channel expression (Ben-Ari 2002). Calcium influx-mediated excitotoxicity has been considered a major cause of neuronal death when neurons are challenged post-TBI with the excitatory amino acid, glutamate (Globus et al. 1995; Saatman et al. 1996). Furthermore, direct application of N-methyl-D-aspartate (NMDA) to hippocampal organotypic slices demonstrated acute excitotoxic injury in the CA1, CA3 and DG regions followed by stimulation of proliferation in the DG, suggesting that TBI-induced vulnerability and subsequent neurogenesis in the DG may, in part, be a consequence of excitotoxic injury (Bunk et al. 2010) . This is consistent with in vivo studies using NMDA receptor antagonists which suppressed injury-neurogenesis in the DG (Bernabeu and Sharp 2000; Arvidsson et al. 2001). Excitotoxicity seemingly overlaps with other types of cell death, such as apoptosis and necrosis, but depends on the intensity threshold of the initiating stimulus. Recent studies indicate that most dying cells in the DG at 24 hours post-moderate TBI did not undergoing apoptosis but instead co-expressed RIP-1, a marker for a subtype of necrotic cell death (Chan et al. 2003). Interestingly, increasing the severity of injury increases apoptotic markers in the GCL (Colicos and Dash 1996; Clark et al. 2001).
Although acute cell loss is observed in the DG after TBI, subsequent recovery or re-population of the injured neurogenic compartment appears to occur in response to cellular loss. Recent findings indicate that the acute cell death, seen within the first week post-injury, of the late DCX-expressing committed neuroblasts can be counterbalanced by the active proliferation of the quiescent type-1 early nestin-expressing progenitors following trauma (Yu et al. 2008) . Indeed, similar to the SVZ response, induction of proliferation is observed in both the contralateral and ipsilateral DG after TBI (Dash et al. 2001; Kernie et al. 2001; Lu et al. 2003; Yoshimura et al. 2003; Emery et al. 2005). Following both CCI and LFP injury models, proliferation in the DG begins as early as 2 days post-injury, peaks during the first week following injury and returns to baseline by 35 days (Rice et al. 2003; Sun et al. 2005). Interestingly, overall cell and new neuron production in the contralateral DG of TBI-injured mice is not significantly different from uninjured controls even though proliferation and DCX-positive cells are increased without any appreciable loss in cell numbers. However, in the ipsilateral hippocampus a significant increase in the number of newly generated cells, expressing neuronal markers, are predominant after longer maturation periods .
The differentiation status of these newly born cells has been examined at several time points after injury (Sun et al. 2005, 2009; Rola et al. 2006; Blaiss et al. 2011). Fate labeling studies indicate that they contribute to TBI-induced neurogenesis in the hippocampus. Previous studies have shown a four-fold increase in the number of BrdU-labeled cells appearing in the SGL at 5 days after injury; however, by 10-weeks only 46 % of them remained in the injured DG compared to 65 % of the originally labeled cells in un-injured mice. This translated into a 2-fold preserved increase in newly born cells in the DG of TBI-injured mice compared to un-injured controls. Double-labeling of the cells indicated that 80 % of the BrdU-labeled cells in the DG expressed NeuN and calbindin, indicative of a mature neuronal dentate phenotype; 10 % were GFAP-positive for astrocytes and the remaining were undergoing cell death (Sun et al. 2007). Similar trends were observed in the control mice, where overall TBI-injured mice had significantly more newly generated neurons and astrocytes as a result of the increased proliferation in the acute phases of injury . No differences were observed in the cell fate. Injection of the retrograde neuronal tracer FluoroGold (FG) into the CA3 hippocampus resulted in greater numbers of FG labeled BrdU-positive cells in the DG at 10 weeks post-injury suggesting connectivity with the CA3 target region. Genetic fate labeling of NSPCs using YFP driven by the nestinδ promoter also showed stable DG neurogenesis at 2 months post-TBI, where YFP-labeled cells persist as NeuN-positive and GFAP-expressing progenitors in the DG (Blaiss et al. 2011) . No YFP-labeled cells were observed as reactive astrocytes in the hilus or DG. Importantly, ablation of TBI-induced neurogenesis following ganciclovir treatment in the nestinδ–HSV–TK transgenic mice exacerbated the cognitive effects of TBI indicating that injury-induced neurogenesis in the DG plays an important role in restoring learning and memory function.
TBI-induced proliferation appears to have a similar temporal trend between the DG and SVZ. However, the DG has a more pronounced neurogenic response to injury as compared to the combined neurogenic and gliogenic response of the SVZ following cortical injury (Kernie et al. 2001). Unlike the SVZ response, NSPCs residing in the injured hippocampus remain localized and active in their neurogenic niche and appear to retain their differentiation and migration patterns during repair. Interestingly, in vitro studies show a similar cell autonomous role between SVZ- and DG-derived NSPCs, where they have similar growth, migration and differentiation potentials in culture . Transplantation of DG-derived NSPCs to the SVZ compartments demonstrates that environmental influences are paramount, since these newly transplanted DG-derived NSPCs migrated to the OB and differentiated into interneurons similar to SVZ-derived NSPCs (Suhonen et al. 1996). Thus, it is thought that differential gene expression within the specialized DG niche, such as those regulating ECM adhesion, cell-cell contacts, chemoattractive and repulsive cues, regulate DG homeostasis and could play important roles in DG-derived NSPCs following TBI. The possibility of enhancing these regulatory cues in the molecular layer of the DG could significantly impact NSPC responses and subsequent cognitive recovery by restoring long-term potentiation and its effects on learning and memory (van Praag et al. 1999; Wang et al. 2005) .
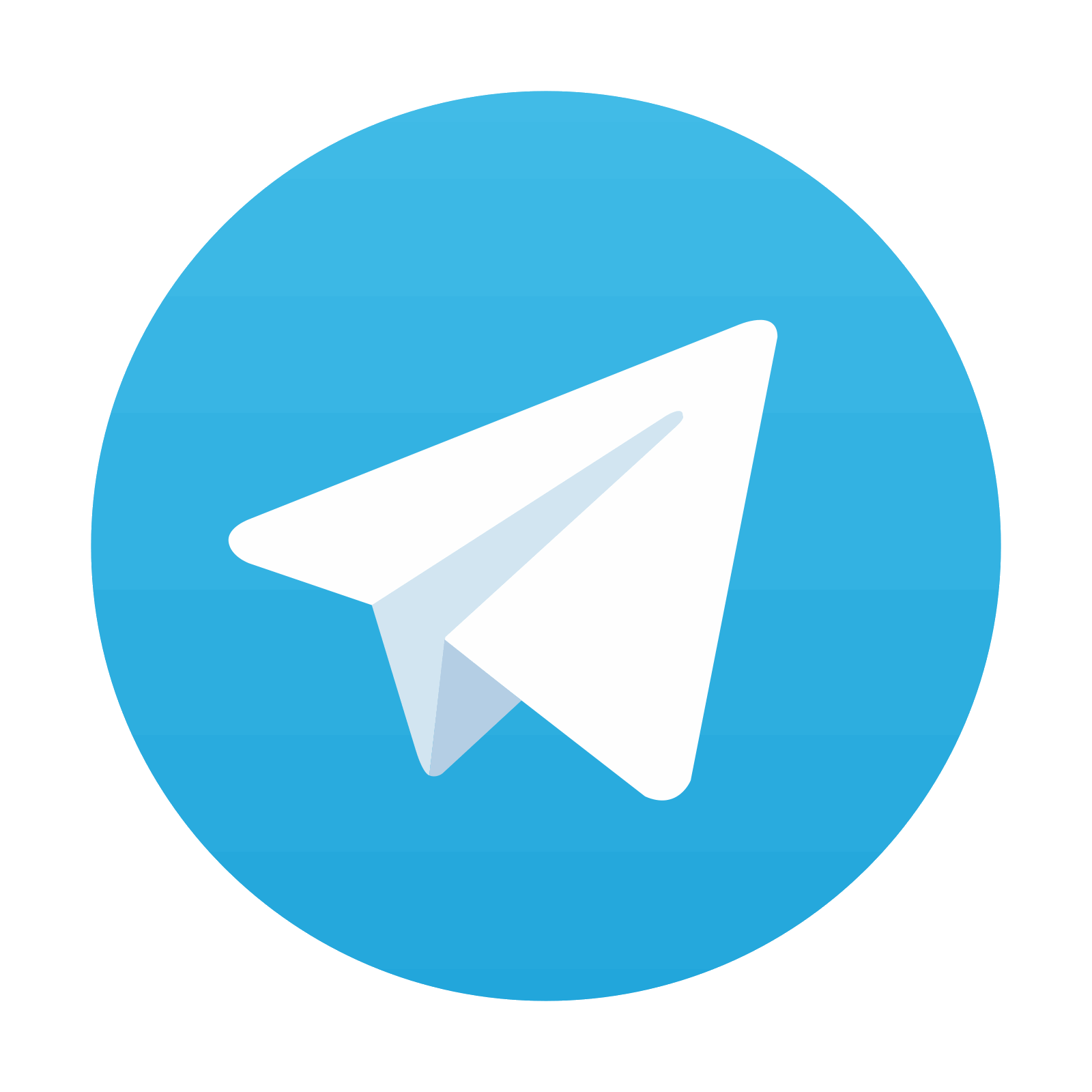
Stay updated, free articles. Join our Telegram channel
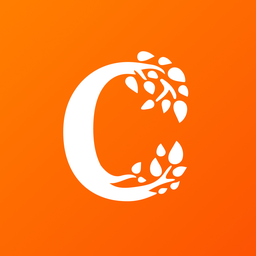
Full access? Get Clinical Tree
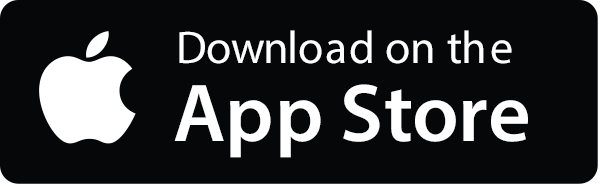
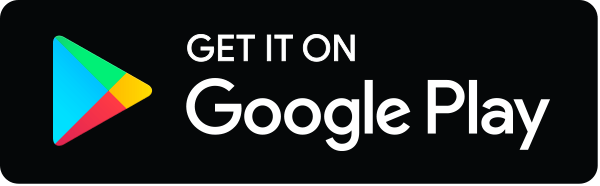