Fig. 7.1
An overview of isolation methods and characteristics of early and late EPCs
7.2.4 Classification of EPCs
According to their culture characteristics and functions, circulation EPCs can be classified into two different populations: early EPCs, which are also called circulating angiogenic cells (CACs), and late EPCs, which are also known as ECFCs (Hur et al. 2004) (Table 7.1) . The early EPCs emerge 5–7 days after isolation of MNCs from the PB and disappear at 4 weeks. They have spindle shape, can be stained with Ulex europaeus agglutinin 1 (UEA−1) and take up acetylated low-density lipoprotein (acLDL) (Hur et al. 2004; Hirschi et al. 2008). They express EC markers and keep hematopoietic antigen expression (Kirton and Xu 2010). Early EPCs cannot form vessels in vivo, but contribute to angiogenesis by secreting angiogenic cytokines (Gehling et al. 2000; Lin et al. 2000; Vaughan and O’Brien 2012). Late EPCs form a monolayer of cobblestone shaped cells 2–4 weeks after plating, have huge potential to proliferate, and can be maintained for up to 12 weeks . Similarly, these cells can also be stained with UEA−1, take up acLDL and express the same markers as early EPCs, such as CD34/KDR (VEGFR−2)/CD31, but they lack the expression of antigens like CD14, CD133, CD45 and CD115 (Hur et al. 2004; Ingram et al. 2004; Kirton and Xu 2010). More importantly, late EPCs are able to form vessels in vitro and in vivo (Lin et al. 2000; Grant et al. 2002). Late EPCs are thought to be the true EPCs and show greater sensitivity to vascular endothelial growth factor (VEGF), basic fibroblast growth factor (bFGF), and placental growth factor (PlGF) (Bompais et al. 2004; Pasquier 2010). Therefore, these two types of EPCs have different morphologies, proliferative abilities and survival rates but both of them display vasculogenic capacity in vivo (Hur et al. 2004) (Fig. 7.1) .
Table 7.1
Characteristics of early and late EPCs
Early EPCs | Late EPCs | Ref | |
---|---|---|---|
Source | PB | BM, PB/CB, vessel well | |
Culture time | 5–7 days | > 14 days | |
Morphology | Spindle | Cobblestone | (Hur et al. 2004) |
Survival fate | Peak growth 2–3 weeks, disappear 4 weeks | Peak growth 2–3 weeks, up to 12 weeks | (Zhao et al. 2013) |
In vitro | Low proliferative ability, no tube-like structure formation | High proliferative ability, capillary-like formation | (Hur et al. 2004) |
In vivo | No vessel formation | Vessel formation | (Hur et al. 2004) |
Function | Cannot regenerate a damaged endothelium | Physically contribute to vascular regeneration | |
Neuro-vasculogenesis | Release angiogenic cytokines | Differentiate into endothelial cells | |
Same surface antigens | CD34/KDR (VEGFR−2)/CD31/CD114/vWF | CD34/KDR (VEGFR−2)/CD31/CD114/vWF | (Kirton and Xu 2010) |
Different surface antigens | CD45/CD14/CD11b/CD115 | CD105/CD146, higher VE-cadherin/KDR |
7.3 Therapeutic Potential of EPCs for Stroke
7.3.1 A Biomarker of Diseases
There is no doubt that EPCs exist in adult PB (Asahara et al. 1997), promote vascular repair after ischemia , and attenuate the progression of arteriosclerosis (Medina et al. 2012). In the past years, a lot of studies have demonstrated that the number and functional stage of circulation EPCs are associated with arteriosclerosis, hypertension, diabetes, and metabolic syndrome (Vasa et al. 2001; Hill et al. 2003; Werner et al. 2005; Liao et al. 2010; Mandraffino et al. 2011; Devaraj and Jialal 2012; Flammer et al. 2012). Based on this evidence, levels of circulation EPCs can be used as novel biomarkers. More importantly, the levels of EPCs also have a close relationship with ischemic stroke, studies have shown that lower levels of circulation EPCs indicate poor outcomes among ischemic stroke patients (Ghani et al. 2005; Sobrino et al. 2007; Chu et al. 2008; Yip et al. 2008; Tsai et al. 2014) (Table 7.2).
Table 7.2
EPCs as a marker for diseases or good outcomes
Diseases | No. patient | EPC phenotype | EPC number | Marker for | Ref |
---|---|---|---|---|---|
IS | 30 | CD34+/KDR+ | ↓ | Increase burden of carotid atherosclerosis | (Lau et al. 2007) |
IS | 48 | CFU-EC | ↑ | Good functional outcome; reduced infarct growth | (Sobrino et al. 2007) |
Stroke | 75-acute 45-chronic | CFU | ↓ | Endothelial dysfunction or repair in acute stroke | (Chu et al. 2008) |
Acute IS | 138 | CD31+/CD34+ CD62E+/CD34+ KDR+/CD34+ | ↑in IS ↓in severe neurological impairment | ↓EPC: severe neurological impairment and adverse clinical outcomes | (Yip et al. 2008) |
CM | 214 | CD34+/VEGFR-2+CD34+/CD133+ | ↓ | A pathophysiologic feature of CM | (Gyan et al. 2009) |
Acute IS | 100 | CD133+/KDR+ | ↓ | Mobilization of EPCs in response to stroke stress | (Zhou et al. 2009) |
Acute IS | 17 | CD34+/CD133+/VEGFR-2+ | ↓ | Larger lesion volumes; acute phase stroke severity | (Bogoslovsky et al. 2010) |
IS | 42 | CD133+/CD34+/KDR+/CD45– | ↑ | Enhanced angiogenic function in the subacute phase of stroke | (Navarro-Sobrino et al. 2010) |
IS | 17 | CD133+/CD34+ | ↓ | Tissue injury and stroke severity in early ischemia | (Bogoslovsky et al. 2011a) |
CA | 27 | CD133+/CD34+ | ↓ | Contributes to the pathophysiological process of aneurysm formation | (Wei et al. 2011a) |
Ruptured CA | 14 | CD133+/CD34+ | ↑ | Prognostic marker for the outcomes of ruptured CA | (Wei et al. 2011b) |
Hypertension | 32 | (CD31+/CD34+/KDR+/CD45– | ↓ | Relates to radiological cerebral small vessel disease manifestations | (Rouhl et al. 2012) |
IAS | 108 | CD34+/CD133+/VEGFR-2+ | ↑ | Independent markers of IAS | (Liu et al. 2013c) |
IS | 146 | CD34+/CD133+/VEGFR-2+ | ↑at day 7 | A better functional outcome | (Marti-Fabregas et al. 2013) |
Acute IS | 65 | CD133+/CD34+; KDR+/CD34+ | ↓ | Poor 6-month outcome in patients with AIS | (Tsai et al. 2014) |
7.3.2 Protection of Blood Brain Barrier (BBB)
As we all know, the BBB is comprised of brain microvascular ECs, basement membrane, astrocytes and pericytes, all of these parts are now called the neurovascular unit (Wong et al. 2013). The integrity of the BBB plays an important role in maintaining the homeostasis of the brain. Once destroyed, the balance of the brain’s microenvironment is disrupted, leading to a series of pathological processes, including the swelling of endothelial cells, an increase in vascular permeability, inflammatory cell infiltration and tissue edema. As mentioned above, EPCs have the potential to differentiate into ECs and promote vascular repair (Ponio et al. 2014), and to support the integrity and function of the BBB (Kaneko et al. 2012). However, how EPCs beneficially influence the BBB is still a mystery. Therefore, more work is needed to elucidate the protective mechanism of EPCs on the BBB after stroke.
7.3.3 Promotion of Neovascularization After Stroke
It has been widely accepted that neovascularization after stroke is essential and critical for tissue repair and neurological function recovery. Animal and human studies have proved that EPCs participate in neovascularization (Zhang et al. 2002; Fan et al. 2010; Paczkowska et al. 2013), mainly via two approaches: by directly differentiating into ECs and incorporating into the damaged vessels, which is called vasculogenesis; and by indirectly promoting migration and proliferation of pre-existing ECs, which is called angiogenesis, through releasing a variety of angiogenic cytokines (Masuda and Asahara 2003; Tepper et al. 2005; Urbich et al. 2005; Chen et al. 2013b). In addition, these cytokines also enhance EC and neuron survival, and recruit endogenous progenitor cells (Chen et al. 2013b). Because of the unique characteristic and advantage of angiogenic ability, EPCs may be an important agent for the treatment of stroke .
7.3.4 Factors Influence EPCs In Vivo
In the past decade, it had been demonstrated that tissue ischemia and exogenous cytokines could mobilize endogenous circulating EPCs and thereby contribute to neovascularization (Asahara et al. 1999; Takahashi et al. 1999). Subsequently, more and more studies have proven that the levels and functional stages of EPCs are correlated with many diseases and are considered as a biomarker (Table 7.2). Moreover, transplantation of EPCs as a therapeutic strategy is beneficial to the hindlimb and cerebral ischemia (Kalka et al. 2000a; Fan et al. 2010; Moubarik et al. 2011). Recently, several studies showed that a variety of factors could influence the number and function of circulating EPCs in vivo. For example, statin treatment for 4 days may increase circulating EPCs levels in acute ischemic stroke patients, probably by nitric oxide (NO)-related mechanisms (Sobrino et al. 2012a). VEGF and stromal derived factor−1α (SDF−1α) are independent factors for the increment of circulating EPCs (Sobrino et al. 2012b). In addition, factors like homocysteine, haptoglobin 1–1, citicoline, cilostazol, systolic blood pressure, total cholesterol, erythropoietin (EPO), high-mobility group box 1 (HMGB−1), and matrix metalloproteinase (MMP−9) are also proven to influence the number of circulating EPCs in humans and animals (Table 7.3). The therapeutic effects of transplantation of EPCs for stroke can be improved by modulating these factors (Morancho et al. 2013).
Table 7.3
Factors that influence EPCs in vivo
Factors | EPC source | Route and time for factors of administration | EPC phenotype | Influence | Patients or animals | Ref |
---|---|---|---|---|---|---|
Homocysteine | PB | — | CD34+/CD31+ | ↑EPCs apoptosis | ? patients with hyperhomocysteinemia | (Alam et al. 2009) |
Haptoglobin 1-1 | PB | — | CD34+/KDR+ | ↓EPCs number | 42 patients with lacunar stroke | (Rouhl et al. 2009) |
BP and total cholesterol | PB | — | CD133+/KDR+ | ↓EPCs number | ? patients with acute stroke | (Zhou et al. 2009) |
SDF-1 | PB | — | CD34+/CD133+/VEGF-R2+ | ↑EPCs number | 17 patients with acute IS | (Bogoslovsky et al. 2011b) |
Citicoline | PB | Oral citicoline (2000 mg/day) | CFU-EC | ↑EPCs number | 48 patients with IS | (Sobrino et al. 2011) |
Cilostazol | PB | 200 mg daily | CD34+/CD133+ | ↑EPCs number | 20 diabetic patients with leukoaraiosis or asymptomatic old cerebral infarction | (Ueno et al. 2011) |
EPO | PB | 200 U/kg 1 month after surgery | CD34+/CD133+ | ↑EPCs number | rat with CA | (Xu et al. 2011) |
EPO | PB | 5000 IU Each time, subcutaneously | CD31+/CD34+, CD62E+/CD34+, KDR+/CD34+ | ↑EPCs number | 167 patients with acute IS | (Yip et al. 2011) |
HMGB-1 | Peri-infarct in brain | — | CD34+/Flk1+ | ↑EPCs number | mice with 45 min MCAO | (Hayakawa et al. 2012) |
Statins | PB | 20 mg atorvastatin/day | CFU-EC | ↑EPCs number | 48 patients with acute IS | (Sobrino et al. 2012a) |
VEGF and SDF-1α | PB | — | CFU-EC | ↑EPCs number | 48 patients with IS | (Sobrino et al. 2012b) |
MMP-9 | Spleens | — | vWF+/ KDR+/CD133+ | Delay EPCs release | Animals with MCAO | (Morancho et al. 2013) |
7.4 Signaling in Regulating EPC Functions
Studies are investigating a variety of factors that influence EPC proliferation, migration and maturation (Table 7.3). Additional researchers are trying to discover the signaling pathways activated by these factors to influence EPCs. Early EPCs secrete a large number of factors, including VEGF, brain-derived neurotrophic factor (BDNF), bFGF, insulin-like growth factor 1 (IGF−1), and interleukin−8 (IL−8). (He et al. 2005; Moubarik et al. 2011; Rosell et al. 2013), which are pro-angiogenic factors that increase endothelial proliferation, tube formation, migration and MMP secretion in ECs to enhance the invasiveness of EPCs (Carmeliet 2003; Li et al. 2003). MMP−9 is essential for ischemia-induced neovascularization, which modulates the neovascularization of EPCs by increasing the release of cytokines (Huang et al. 2009; Morancho et al. 2013). Integrin-linked kinase is upregulated in ECs and associated with increased intercellular adhesion molecule 1 (ICAM−1) and SDF−1 under hypoxic stress, which recruits EPCs to ischemic tissue (Lee et al. 2006). CD18 and its ligand ICAM−1 also play an essential role in mediating EPC recruitment in infracted hearts (Wu et al. 2006). Activated AKT signals promoted the expression of ICAM−1 on ECs and closely associated with EPC entrapment, which might be important in regulating the process of neovascularization through enhancing EPC migration and trans-endothelial migration (Yoon et al. 2006; Hur et al. 2007).
IL−10 increases EPC survival and mobilization through the activation of STAT3/VEGF signaling cascades (Krishnamurthy et al. 2011). SDF−1 released from the ischemic tissue form a concentration gradient to promote EPC homing through interaction with its receptor CXCR4 (Fan et al. 2010). Deltalike−4 gene modified EPCs show enhanced functional neovascularization in ischemic tissue due to the activation of Notch/Hey1/mTOR/p70S6K signaling pathways (Huang et al. 2013a). Wnt1 is a pro-angiogenic molecule and enhances EPC function in a hepatocyte growth factor (HGF)-dependent manner (Gherghe et al. 2011). HMGB1 secreted by astrocytes after ischemic stroke increases EPC homing involved in neurovascular remodeling and functional recovery (Hayakawa et al. 2012). Other factors have also been reported to influence EPC functions, such as E-selectin, estrogen and β2-adrenergic receptor (Oh et al. 2007; Tan et al. 2012; Galasso et al. 2013). Fully understanding the mechanisms underlying EPC function will help improve the safety and efficiency of EPC transplantation.
7.5 Action Mechanism of EPCs
7.5.1 Cell Replacement
EPCs derived from BM or other tissues have an intrinsic capacity for differentiating into ECs (Asahara et al. 1997; Beltrami et al. 2003; Planat-Benard et al. 2004; Chen et al. 2008; Chen et al. 2012; Nih et al. 2012; Iskander et al. 2013; Pellegrini et al. 2013). The injured ECs in the brain can be replaced by transplanted EPCs. Granulocyte colony-stimulating factor (G-CSF) mobilizes circulating EPCs to engage 39 % of the total luminal length of the neoendothelium (Takamiya et al. 2006). LacZ-transduced CD34+EPC transplantation leads to about 60 % reendothelialization of balloon-injured rabbit carotid arteries costained with CD31 as early as 4 days after transplantation and this increases to about 70 % at 30 days after transplantation (Griese et al. 2003b). Fluorescence-labeled EPCs are found in the neointima and costaining with vWF is found after 4 weeks in the injured carotid artery of balloon injured New Zealand white rabbits (Hu et al. 2013). Hence, cell replacement is one of the mechanisms of vascular repair by progenitor cells. BM derived EPCs contribute to the microvascular structure of the choroid plexus by differentiating into ECs during cerebral ischemia in adult mice (Zhang et al. 2002). 14 days after the transplantation of EPCs in the cerebral ischemia rabbit model, a decrease in the number of apoptotic cells and an increase in the microvessel density in the ischemic boundary area has been witnessed, and most of EPCs capable of binding to UEA−1 lectin are incorporated into capillaries (Chen et al. 2008).
However, the extent of incorporation of BM derived cells in cerebral vessels after stroke has varied in previous studies (Hess et al. 2002; Zhang et al. 2002; Machein et al. 2003; Chen et al. 2008; Moubarik et al. 2011). Whereas positive vessels had an average of 34 % endothelial marker expressing BM derived cells (Hess et al. 2002; Zhang et al. 2002), others could not detect endothelial marker expressing cells (Machein et al. 2003; Moubarik et al. 2011).
7.5.2 Enhanced Trophic/Regenerative Support for Endogenous Repair Processes
Neovascularization is not solely the result of the incorporation of EPCs in newly formed vessels; the release of trophic factors in a paracrine manner may also influence neovascularization. Cultured PB MNCs secrete high levels of VEGF, HGF, G-CSF and granulocyte-macrophage colony-stimulating factor (GM-CSF) (Rehman et al. 2003). More and more researchers are paying close attention to the trophic effects of EPCs. In vitro, early EPCs cultivated from different sources have shown marked expression and the release of angiogenic cytokines including G-CSF, GM-CSF, VEGF, platelet-derived growth factor (PDGF), epidermal growth factor (EGF), FGF, HGF, IL−8, transforming growth factor β2 (TGF-β2), IGF−1 and etc (He et al. 2004; Hur et al. 2004; Yoon et al. 2005). The release of these growth factors in turn may influence the classical process of angiogenesis, particularly the proliferation and migration as well as the survival of mature ECs (Folkman 1995; Urbich and Dimmeler 2004). EPCs can also exert a strong mitogenic effect on mature ECs and enhance the angiogenic capacity of outgrowth of ECs via secretion of IL−8 with/without VEGF (He et al. 2005; Yoon et al. 2005).
In cerebral arteries, the paracrine effect of EPCs promotes vasoprotection by increasing prostacyclin production and the intracellular concentration of cAMP (Santhanam et al. 2007). EPCs from stroke patients present higher levels of pro-angiogenic factors at early stages, which decrease in mature ECs when they become more similar to mature microvascular ECs (Navarro-Sobrino et al. 2013). 24 h after the administration of EPCs expressing GFP, they are found to express endothelial NO-synthase (eNOS) and distribute in the brain parenchyma and around the endothelial layer of pial arteries in the ischemic lesions (Ohta et al. 2006). EPC transplantation can also induce humoral effects, which are sustained by host tissues, decrease apoptosis and augment proliferation of cells. Transplantation of EPCs enhances the mobilization of endogenous EPCs and HSCs mainly by upregulation of humoral VEGF, FGF−2, IGF, HGF, angiopoietin−1 and SDF−1 (Cho et al. 2007).
Studies have shown that vascular niche can support neurogenesis in the subventricular zone and the dentate gyrus by secreting growth factors associated with neurogenesis, such as VEGF or BDNF (Leventhal et al. 1999; Palmer et al. 2000). In an experimental stroke study, neovascularization related to neurogenesis, and also to the migration of neural progenitor cells (NPCs), along the newly formed vessels (Thored et al. 2007). Thus, administered EPCs may enhance the proliferation of endogenous NPCs in the brain (Rouhl et al. 2008). EPCs injected 24 h after MCAO were found in the injured area and improved functional recovery, which was linked to a reduction in ischemia-induced apoptosis and a stimulation of ischemia-induced angiogenesis and neurogenesis (Moubarik et al. 2011). Transplantation of BM-derived EPCs exerts potent neuroprotective functions against cerebral ischemia/reperfusion injury in rats, and the protective effects may be associated with decreased expression of Bax, caspase−3 and p67phox and the increasing expression of Bcl−2 and manganese superoxide dismutase (MnSOD), which promotes anti-oxidative and anti-apoptotic properties (Qiu et al. 2013).
7.6 Transplantation of EPCs in Ischemic Stroke Animals
7.6.1 Transplantation Routes for EPCs
The optimal transplantation route for EPCs following ischemic stroke may be important for the therapeutic efficacy . The two routes mostly used for the transplantation of EPCs in stroke are intracerebral and intravascular injections. They each have their own advantages and disadvantages. EPCs intracerebrally injected into the peri-infarct area may be immediately involved in incorporating newly formed vessels or secreting trophic factors to support endogenous repair processes, especially in permanent ischemic stroke to bypass the occlusion of blood vessels. However, invasive injury to the brain raises safety issues.
Intravascular injection either through veins or arteries has minimal invasive injury potential for systematical cell distribution, as well as the far-flung secretion of neuroprotective, pro-angiogenic and immunomodulatory factors (Misra et al. 2012). Intravenously grafted cells can follow a chemokine generated gradient formed by the injured brain and penetrate through the BBB (Guzman et al. 2008), and grafted cells do not have to be near the lesion to be effective (Borlongan et al. 2004) . However, very few cells have been found to integrate into the infarct area. The majority of cells became stuck in the lung, liver, and spleen after intravenous administration. Intra-arterial delivery, in contrast, overpasses the peripheral filtering organs, leading to higher cell engraftment to the brain (Li et al. 2010; Zhang et al. 2012), and greater efficacy (Kamiya et al. 2008; Pendharkar et al. 2010). There is a concern that intra-artery transplanted cells can stick together and cause microemboli, including lethal pulmonary emboli or a reduction in cerebral blood flow, which is associated with microstrokes (Walczak et al. 2008).
In preclinical experimental stroke, intravascular injections are usually used, and they are applied through the tail vein (Zhang et al. 2002; Chen et al. 2012; Nih et al. 2012; Chen et al. 2013d; Decano et al. 2013; Qiu et al. 2013), femoral vein (Moubarik et al. 2011; Pellegrini et al. 2013), jugular vein (Fan et al. 2010; Li et al. 2013), and internal carotid artery (Ohta et al. 2006) (Table 7.4) . Despite the different routes used for EPC transplantation, decreased infarct volume, improved neurobehavioral outcomes, increased angiogenesis and neurogenesis, attenuation of endothelial dysfunction, even anti-apoptosis effects have been observed during study. These studies may benefit from both functions of EPCs during cell replacement and enhanced trophic/regenerative support for endogenous repair processes.
Table 7.4
Preclinical EPC transplantation studies
Animal models | EPC source | EPC phenotype | Modification | Co-transplantation or not? | Dose | Route and administration time | Outcomes | Mechanisms | Ref |
---|---|---|---|---|---|---|---|---|---|
Embolic stroke in mice | BM | NM | No | No | 2 × 106 | IV via tail vein, 4 weeks before stroke | ↑neovascularization | ↑angiogenesis and vasculogenesis | (Zhang et al. 2002) |
tMCAO in rats | BM | NM | No | No | 2.5 × 105 | IA, immediately after stroke | ↓infarct volume and neurological deficits | ↑eNOS, VEGF, IGF-1; ↓endothelial dysfunction | (Ohta et al. 2006) |
pMCAO in rabbits | BM | UEA-1, acLDL | No | No | 3 × 107 | IV, 1 day after ischemia | ↓infarct volume and neurological score | ↓tunel+ cells; ↑vessel density↑angiogenesis | (Chen et al. 2008) |
1 h tMCAO in mice | PB | KDR+/VE-cadherin+/vWF+/Tie−2+; UEA, acLDL | No | No | 1 × 106 | IV via left jugular vein, 1 h after tMCAO | ↓ischemic infarct volume and neurological deficits | ↑angiogenesis | (Fan et al. 2010) |
tMCAo in rats | PB | CFU-EC | No | No | 4 × 106 | IV via femoral vein, 1 day after MCAO | ↓apoptosis and reactive astrogliosis; ↑capillary density and neurogenesis | ↑IGF-1, VEGF secretion | (Moubarik et al. 2011) |
pMCAO in type 2 diabetic mice | BM | CD34+/VEGFR-2+/lectin+; acLDL | CXCR4 gene modified | No | 2 × 105 | IV via tail vein, 2 h after MCAO | ↑CBF; ↓infarct volume and neurological score | ↑angiogenesis and neurogenesis | (Chen et al. 2012) |
Distal MCAO in mice | UCB | CD34+ | No | SMPCs, 2.5 × 105 | 2.5 × 105 | IV via tail vein, 1 day after MCAO | ↑angiogenesis, vascular remodeling | maintenance of neurogenesis and neuroblast migration | (Nih et al. 2012) |
TBI in rats | Spleens | NM | Labeled with SPIO | No | 106 | IV via tail vein, at 6–12 h after TBI | ↑CBF | ↑microvascular density | (Chen et al. 2013d) |
Stroke-prone Rats | PB | CD45–/CD34+/KDR+ | No | No | NM | IA via tail vein, — | Attenuated progression and delayed stroke onset | Maintain vascular healthy | (Decano et al. 2013) |
tMCAO in rat | UCB | CD133+ | No | No | 1 × 107 | IV, 1 day after tMCAO | ↓infarct volume | ↑endogenous proliferation, angiogenesis, and neurogenesis | (Iskander et al. 2013) |
tMCAO in rat | UCB | CFU-EC | No | EPO (2500 UI/kg per day × 3) | 5 × 106 | IV via femoral vein, 1 day after tMCAO | ↓apoptosis; ↑angiogenesis and neurogenesis | (Pellegrini et al. 2013) | |
tMCAO in rat | BM | CD31+/CD34+/CD133+/Flk-1+ | No | No | 106 | IV via tail vein, immediately and 12 h after reperfusion | ↓I/R injury | ↓caspase-3 activity, Bax and NF-κB, ↑Bcl-2 expression; anti-oxidative and anti-apoptotic properties | (Qiu et al. 2013) |
However, when considering application in clinical trials , the routes of transplantation of EPCs should be standardized to ease administration. Several clinical studies have been carried out as illustrated in Table 7.5. These studies look into the safety and efficiency of routes for EPC transplantation in human patients, and there is still a lot of work to do in this field .
Table 7.5
Studies on clinical EPC transplantation
Phase | Patients’ symptoms | No. patients | EPC source | EPC markers | Dose | Route | Outcome measures | Status | Location | NTC identifier |
---|---|---|---|---|---|---|---|---|---|---|
I/II | 18–80 years, ≤ 7 days of after stroke; NIHSS ≥ 7 at day 7 | NM | BM | 2.5 × 106/kg | IV | Number of adverse events; Changes in functional outcomes | Recruiting | China | NCT01468064 | |
I | 35–75 years, ≥ 6 and ≤ 60 months after stroke | NM | UCB | CD34+ | 2–8 × 106/patient | IC | Change from baseline in NIHSS and brain Image | Not yet recruiting | China | NCT01438593 |
II | 35–70 years, ≥ 6 and ≤ 60 months after stroke, NIHSS (9–20) | 30 | PB | CD34+ | 2–8 × 106/patient | IC | NIHSS, European stroke scale, Barthel index and Mini-Mental State Examination, MRI and CT scans | Completed | China | NCT00950521 |
I/II | 30–80 years, ≤ 7 days of onset, NIHSS ≥ 8 | 10 | BM | CD34+ | NM | IA | Safety, Modified Rankin Score, NIHSS | Recruiting | United Kingdom | NCT00535197 |
— | ≥ 30 years, ≥ 6 months and ≤ 5 years after stroke, NIHSS (9–20) | 36 | PB | CD34+ | Plus G-CSF | IC | Not mentioned | Unknown | China | NCT01239602 |
7.6.2 Modification of EPCs
7.6.2.1 Gene Modification
Considering the paracrine-mediated mechanisms of EPCs, the enhancement of their secretion of trophic factors capacity by the overexpression of related genes would be valuable to magnify the efficacy of EPC therapies in stroke treatment (Chen et al. 2013a). EPCs have been modified by a variety of genes before transplantation and have been reported to enhance functional recovery, these genes include VEGF (Asahara 2007; Gou et al. 2011; Yang et al. 2012), HGF (Song et al. 2009), IGF−1 (Sen et al. 2010), paraoxonase−1 (Wang et al. 2010), CXCR4 (Chen et al. 2012), SDF−1 (Schuh et al. 2012), NO (Chen et al. 2013c), home oxygenase−1 (Long et al. 2013), hypoxia-inducible factor−1α (HIF−1α) (Jiang et al. 2008) and Deltalike−4 (Huang et al. 2013a). There are two major methods for gene transfer systems, viral and nonviral. The most widely used viral vectors for gene transfer are adenovirus and retrovirus. Nonviral methods include the introduction of naked DNA into the target cells and the use of liposomes (Vale et al. 2001).
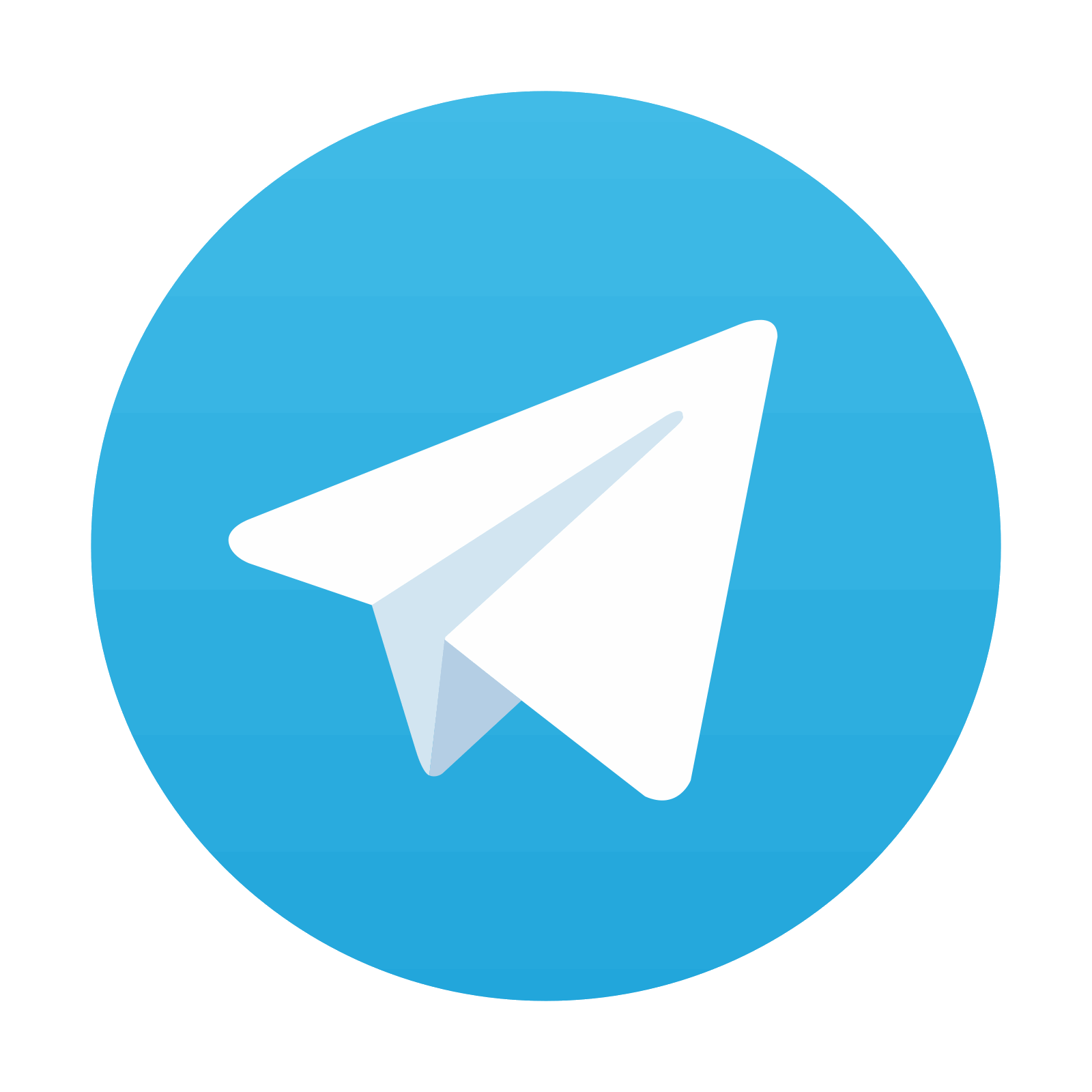
Stay updated, free articles. Join our Telegram channel
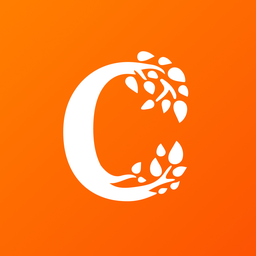
Full access? Get Clinical Tree
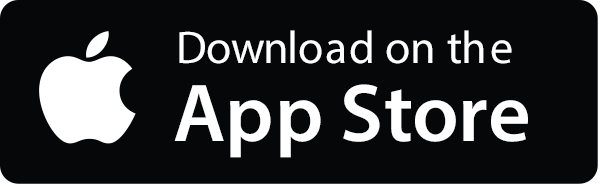
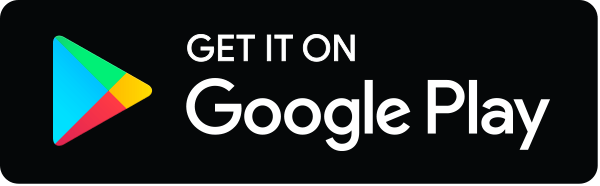