Fig. 8.1
Schematic illustration of selected endothelium-dependent effects that are present under normal conditions. Endothelial NO synthase (eNOS) produces NO under basal conditions, and in response to a variety of receptor-mediated agonists or increased shear stress. eNOS-derived NO produces relaxation of vascular muscle by activating soluble guanylate cyclase (sGC) causing production of cGMP from GTP. In addition to effects on vascular tone, NO inhibits thrombosis via inhibitory effects on platelets and other mechanisms. NO also inhibits vascular inflammation via effects on nuclear factor κB, endothelial adhesion molecules, etc. Cyclooxygenase (COX) produces PGH2 which can then be converted into prostacyclin (PGI2) by prostacyclin synthase or thromboxane A2 by thromboxane synthase. PGI2 also inhibits aggregation of platelets thus suppressing thrombosis. COX also produces superoxide (O2 −) during the metabolism of arachidonic acid. Superoxide is converted into H2O2 by superoxide dismutase (SOD). H2O2 can function as an endothelium-derived relaxing factor (EDHF) producing relaxation of underlying vascular muscle via effects on K+ channels. Some studies suggest that K+ may also function as an EDHF. Lastly, hyperpolarization that is initiated within endothelial cells (endothelium-dependent hyperpolarization, EDH) may spread to vascular muscle via myoendothelial gap junctions without the release of an EDHF. ADMA asymmetric dimethylarginine, BH 4 tetrahydrobiopterin, Cav-1 caveolin-1
3 NO Biology
It is well established that endothelium-derived vasoactive factors are crucial regulators of cerebral vascular tone [17]. Once released from the endothelium, these factors cross the subendothelial space and ultimately cause vasodilation or vasoconstriction. Since its discovery as an EDRF in the aorta, NO has been extensively studied and it has become apparent that NO is central to the regulation of vascular tone in many vascular beds including large and small vessels in both animal models and people.
NO is generated by NO synthase (NOS) enzymes. Three isoforms of NOS have been identified, namely inducible NOS (iNOS or NOS1), neuronal NOS (nNOS or NOS2), and endothelial NOS (eNOS or NOS3). iNOS is an inducible, Ca2+-independent enzyme which generates large amounts of NO upon activation [24, 25]. In contrast, eNOS and nNOS generate relatively smaller amounts of NO and their activity is Ca2+-dependent [24, 25]. Both eNOS and nNOS are constitutively expressed in blood vessels in endothelium and perivascular neurons (respectively), whereas iNOS is not expressed under normal conditions in vascular cells. However, expression of iNOS may be induced in the vessel wall in response to a variety of inflammatory stimuli and in disease [26–28]. All NOS isoforms catalyze the reaction between l-arginine and molecular oxygen via a two-step process involving the generation of the unstable intermediate N-hydroxy-l-arginine before ultimately producing NO and l-citrulline [29, 30]. This process involves the presence of nicotinamide adenine dinucleotide phosphate (NADPH), tetrahydrobiopterin (BH4), flavin adenine dinucleotide (FAD), and flavin mononucleotide. Once generated by NOS, NO can rapidly diffuse to other cells where its main target is the cytosolic enzyme, soluble guanylate cyclase (sGC). Upon activation, sGC catalyzes the conversion of guanosine triphosphate (GTP) to cyclic guanosine monophosphate (cGMP), which serves as an important second messenger, mediating most of the vascular effects of NO. cGMP has multiple downstream targets including cGMP-dependent protein kinases (cGKs), phosphodiesterases, and cGMP-modulated cation channels [31, 32]. Activation of cGKs and cGMP-modulated cation channels can lead to a decrease in intracellular Ca2+ and thus cause vasodilation.
Many studies have shown that activation of eNOS and/or the sGC–cGMP pathway leads to cerebral vasodilation [33–35]. This signaling cascade is active under basal conditions, thus influencing resting vascular tone. For example, inhibitors of NOS cause constriction of cerebral arteries, pial arterioles, and parenchymal arterioles resulting in increases in vascular tone and decreases in cerebral blood flow in diverse experimental models and in humans [36–42]. Such findings, along with other lines of evidence indicate that constitutive activity of NOS and levels of NO in the cerebral vasculature are sufficient to influence vascular tone and thus cerebral blood flow under normal conditions.
Endothelium-derived NO mediates responses of cerebral blood vessels to neurotransmitters including acetylcholine and substance P, metabolic factors like insulin and adiponectin, and therapeutic agents such as HMG-CoA reductase inhibitors (statins) [11, 42, 43]. For example, dilation of small cerebral arterioles to acetylcholine is mediated by activation of type-5 muscarinic receptors and eNOS [35, 44]. In addition to these effects, endothelial cells play a critical role in neurovascular coupling [45]. Recent work has shown that selective inhibition of endothelial signaling prevents retrograde (upstream) dilation of cerebral arterioles on the brain surface in response to activation of the somatosensory cortex [45]. In regions such as the basal forebrain, neurovascular coupling is mediated by neuronally released acetylcholine acting on endothelium with subsequent activation of eNOS (see [11]). These mechanisms of blood flow regulation are important for multiple reasons including the fact the basal forebrain is a key region in relation to the development of dementia and Alzheimer’s disease, processes that are accelerated in the presence of hypertension. Other lines of evidence also support a role for eNOS in neurovascular coupling [46].
In addition to its effects on cerebrovascular tone, NO has a significant influence on the structure of cerebral arteries and arterioles [47]. In mice for example, genetic deletion of eNOS or chronic treatment with an inhibitor of NOS produces hypertrophy of cerebral arterioles and rarefaction of collateral arterioles [47, 48]. Lastly, NO inhibits the aggregation of platelets and adhesion of leukocytes to the vessel wall as well as suppressing the metabolism of amyloid precursor protein, a key molecule in the pathogenesis of Alzheimer’s disease [11, 49–51]. Thus, it is clear that NO is central to the regulation of cerebrovascular homeostasis in both large and small vessels.
4 Free Radical Biology, Oxidative Stress, and Endothelial Dysfunction
Reactive oxygen species (ROS) are primarily formed as by-products of cellular metabolism or by specialized oxidases. Common ROS in cells and tissues include superoxide, hydrogen peroxide, and hydroxyl radical. ROS typically oxidize or reduce other molecules and thereby can modify the activity and structure of proteins, lipids, and small molecules including NO [52–55]. Oxidized proteins and lipids may also serve as second messengers in signaling cascades [52].
There are many potential sources of ROS within the cell including the mitochondrial respiration chain [56], xanthine oxidase [57], cyclooxygenases [58, 59], and importantly the family of NADPH oxidases [9, 60, 61]. NADPH oxidases are probably the most studied family of oxidases in the vasculature, and are associated with the modulation of multiple processes including inflammatory responses, cell growth and proliferation, and cellular metabolism [55]. Furthermore, the NADPH oxidase family of enzymes is currently the only known enzymes whose sole purpose is to generate ROS [60]. The other enzymes listed above generate ROS as a by-product of normal metabolism or during a dysfunctional state [62]. Therefore, NADPH oxidases are prime candidates for generating the excessive levels of ROS that has been described during disease, including hypertension. In summary, ROS derived from oxidases modulate multiple cellular processes and exert important effects on vascular homeostasis.
Cells have developed sophisticated systems to regulate levels of ROS both intracellularly and extracellularly, as well as in specific subcellular compartments. Superoxide is readily metabolized by superoxide dismutase (SOD) to hydrogen peroxide. Three isoforms of SOD exist: cytoplasmic (CuZnSOD, SOD1), mitochondrial (MnSOD, SOD2), and extracellular (EcSOD, SOD3) [63, 64]. Hydrogen peroxide is also metabolized by specific enzymatic systems that include catalase and several isoforms of glutathione peroxidase. Enzymatic antioxidant systems are complex, and cooperate with nonenzymatic antioxidants such as vitamin C and vitamin E [65].
Cellular metabolism is adapted to the redox state in cells. Redox state is determined by the balance of oxidative and reductive reactions, and the activity of antioxidant systems described above [66]. This redox state may vary according to the function and biological state of cells and tissues [67]. When the balance of oxidants and antioxidants is lost, the redox state of cells change, and oxidative stress ensues.
Oxidative stress affects vascular physiology and contributes to vascular pathophysiology. NO signaling and metabolism is extremely sensitive to oxidative stress. Oxidation of BH4 by free radicals can impair the function of NOS resulting in the generation of superoxide rather than NO (termed NOS uncoupling) [11, 13]. Although uncoupled eNOS is thought to be a source of superoxide and oxidative stress in some portions of the circulation [53, 68], the overall importance of this mechanism in the cerebral circulation is still unclear. Importantly, superoxide readily reacts with NO to form the reactive nitrogen species (RNS) peroxynitrite [69]. Peroxynitrite reacts with aromatic amino acids in proteins to form nitrotyrosine adducts and that can then modify protein function [54, 69, 70]. Thus, an oxidative environment may change the character of NO metabolism, decreasing NO bioavailability in blood vessels, and promoting further oxidative damage by NOS uncoupling and/or increased formation of RNS. Although the result of these reactions for the vessel can be quite diverse, one of the most studied consequences is impairment of endothelium-dependent vasodilation [11, 69].
5 Hypertension and Cerebrovascular Function
Hypertension affects cerebrovascular structure and function through a number of mechanisms. For example, impairment of endothelium-dependent vasodilator responses are described in many models of hypertension including angiotensin-II (Ang-II)-dependent models, the spontaneously hypertensive rat (SHR), and the stroke-prone SHR (SHRSP) (Table 8.1). Although perhaps the best described mechanism for endothelial dysfunction involves loss of NO bioavailability, endothelial dysfunction extends to other mechanisms as well including NO-independent pathways and generation of EDCFs.
Table 8.1
Studies of endothelium-dependent responses in experimental models of hypertension
Model |
---|
Acute hypertension [158] |
Angiotensin-II-dependent hypertension |
Genetic (R+A+) [79] |
DOCA-salt (nephrectomy) [93] |
DOCA-salt (no nephrectomy) [94] |
Genetic models |
Intermittent hypoxia [161] |
Aldosterone [162] |
L-NAME [163] |
Aortic banding [164] |
Loss of NO bioavailability most often results from an imbalance between ROS generation and ROS degradation or metabolism (oxidative stress). As the reaction between NO and superoxide is one of the fastest known in biology [71], increased superoxide will reduce NO levels if superoxide is present in close proximity to the NO molecule. Numerous studies have demonstrated that NO-dependent vasodilation (NO-dependent signaling) is impaired during hypertension and this change is thought to be primarily due to scavenging of NO by superoxide resulting in an acute reduction in NO bioavailability.
5.1 Ang-II-Dependent Models of Hypertension
Endothelium-dependent vasodilation is reduced in models of Ang II-dependent hypertension. Most of this work has been done using mice in which hypertension is induced by chronic systemic administration of Ang-II—typically for a few weeks. Although effects on NO-dependent responses are often described in these models, impaired vasodilation can also occur via other endothelium-dependent mechanisms. Reduced NO bioavailability is closely associated with elevated ROS levels. Numerous studies have reported that Ang-II increases superoxide generation in the cerebral vasculature [9, 72–75] and as described above, is likely to scavenge NO, thus reducing its bioavailability.
It has been shown that endothelium-dependent vasodilation is impaired in isolated cerebral arteries following either acute or chronic Ang-II administration as well as in mice with lifelong (genetic) elevations in Ang-II [9, 76–80]. Endogenous NO-dependent vasodilation, as measured by responses to endothelium-dependent vasodilators such as acetylcholine and bradykinin, are significantly impaired in vessels from hypertensive animals compared with controls. Importantly, vasodilation to exogenously administered NO is similar between normotensive and hypertensive animals indicating that vascular muscle can respond normally. An oxidative stress-dependent mechanism accounts for reduced endothelium-dependent vasodilation as treatment with scavengers of superoxide typically restores endothelial function largely to normal [9, 76, 79].
There has been much interest in identifying the underlying cause or source of oxidative stress during hypertension. As discussed above, NADPH oxidases have been postulated to be a major source of elevated ROS levels during disease. Several studies have examined the importance of Nox2 in relation to acute and chronic effects of Ang-II on cerebrovascular function (Fig. 8.2) [8, 9, 69, 72, 73]. For example, a recent study used mice genetically deficient in either Nox1 or Nox2 NADPH oxidase to determine the source of augmented ROS during chronic Ang-II infusion. Following treatment with Ang-II, Nox2-deficient mice were protected against endothelial dysfunction as measured by dilation of basilar arteries to acetylcholine [78]. Interestingly, a role for Nox1 NADPH oxidase was also observed as dilation to acetylcholine was improved compared with arteries from wild-type mice; however, the improvement was less than what was observed in Nox2-deficient mice [78]. Thus, these findings would indicate that the impaired endothelial function in Ang-II infusion models of hypertension is dependent on oxidative stress with contributions by multiple Nox isoforms although Nox2-containing NADPH oxidase appears to be of greatest importance. In relation to signaling pathways, other evidence indicates that activation of signal transducer and activator of transcription 3 (STAT3) plays an important role in these processes. Small molecule inhibitors of STAT3 activation protect against Ang-II-induced oxidative stress and cerebrovascular endothelial dysfunction [81].
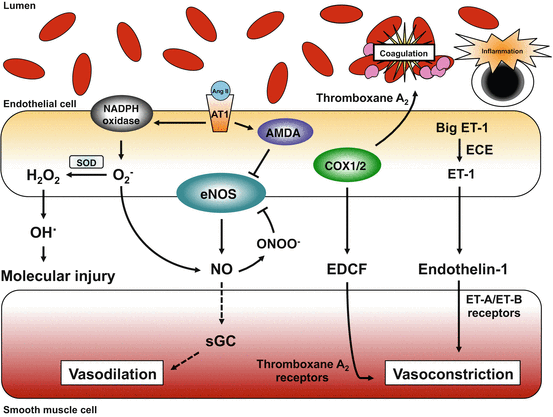
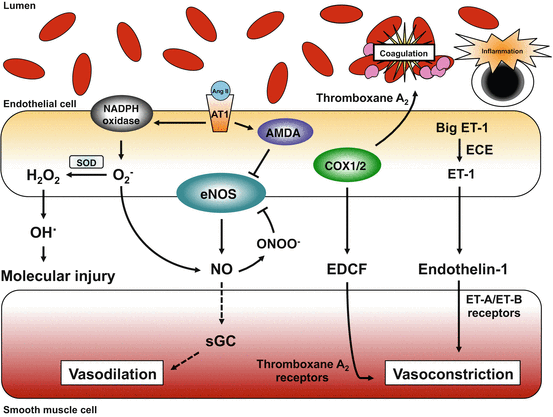
Fig. 8.2
During hypertension and other risk factors for vascular disease, many changes occur within endothelial cells that result in increased vascular tone as well as a prothrombotic environment and low-grade inflammation. There are multiple sources of superoxide (O2 −) in endothelium and vascular muscle (not shown). A major source of O2 − in vascular cells during hypertension is NADPH oxidase. When activated by angiotensin-II (Ang II) acting on AT1 receptors (or other stimuli), O2 − can interact with NO or be converted into other reactive oxygen species including hydroxyl radical (HO.) which is highly reactive and induces mutations in nucleic acids, lipid peroxidation, protein damage, etc. The interaction between O2 − and NO not only reduces NO bioavailability and NO signaling (less activation of sGC), but also results in the formation of peroxynitrite (ONOO−). ONOO− has direct effects of its own but can also reduce formation of NO from eNOS, via effects on tetrahydrobiopterin (BH4). During vascular disease, production of thromboxane A2 and endothelin-1 (ET-1) are increased, contributing to increased vascular tone and platelet aggregation. ECE endothelin-converting enzyme
In addition to studies of isolated cerebral arteries, numerous studies have investigated the effect of hypertension on smaller cerebral arterioles or on changes in local cerebral blood flow in vivo [8–10, 69, 73, 80, 82, 83]. In general, findings obtained using these approaches are similar to those observed in larger cerebral arteries—both acute and chronic Ang-II-dependent hypertension [76] resulted in impairment of endothelial- and NO-dependent vasodilator responses in hypertensive animals compared with controls. Vasodilation to exogenous NO donors and other agonists that act directly on vascular muscle are typically comparable between normotensive and hypertensive animals. In these studies of the microcirculation, oxidative stress was again identified as the key mechanism underlying impaired vasodilator responses as ROS scavengers typically restored endothelium-dependent regulation of vascular tone largely to normal [9, 73, 84]. Importantly, studies using pharmacological inhibitors and genetically modified mice identified Nox2 NADPH oxidase as the source of superoxide [9, 73]. Interestingly, when hypertension was induced by acute administration of the α1-adrenoceptor agonist phenylephrine, endothelium-dependent responses were not impaired [10] suggesting that Ang-II-induced dysfunction is dependent on direct effects of Ang-II and not elevations in blood pressure per se. Consistent with this concept, chronic infusion of a non-pressor dose of Ang-II, or local treatment with Ang-II, also produced endothelial dysfunction in cerebral blood vessels [10, 76]. In relation to this latter point, it has been reported that a dose of Ang-II that elevates blood pressure more gradually (i.e., over a 7-day period vs. ~2–3 days for higher doses of Ang-II) also has profound effects on cerebrovascular function. Like the use of higher doses, this slow-pressor dose of Ang-II promotes oxidative stress and endothelial dysfunction (based on measurements of local cerebral blood flow) before the onset of hypertension [82]. This study also further highlights that many of the effects of hypertension on the cerebral circulation are due to direct effects of Ang-II on cerebrovascular function.
Transgenic mice that genetically express human renin and human angiotensinogen (R+A+ mice) represent a model of truly chronic (lifelong) Ang-II-dependent hypertension. Consistent with data obtained where mice were infused with Ang-II for up to a few weeks, marked superoxide-mediated endothelial dysfunction is present in the basilar artery of these mice [79]. In relation to effects of hypertension on vascular structure, both inward microvascular remodeling and vascular hypertrophy occur in small cerebral arterioles in R+A+ mice [85]. Both pressor and non-pressor doses of Ang-II increased superoxide and produced inward remodeling of cerebral arterioles in wild-type mice, effects that were absent in mice lacking the Nox2 component of NADPH oxidase [75]. These studies provide direct evidence that NADPH oxidase was a key source of superoxide that is required for Ang-II-induced inward remodeling to occur in cerebral arterioles.
In summary, findings to date indicate that in Ang-II-dependent models of hypertension, there is increased oxidative stress within the cerebral vasculature. Elevated superoxide levels overwhelm antioxidant defense mechanisms resulting in impairment of NO-dependent vasodilation, a major signaling pathway for endothelium-dependent effects on vascular muscle and other cell types. Furthermore, Ang-II and superoxide contribute to alterations in cerebrovascular structure, which can further compromise the function of the cerebrovasculature. These effects are likely to have important consequences for regulation of cerebral blood flow.
5.2 Deoxycorticosterone (DOCA)/Salt-Dependent Hypertension
Chronic administration of DOCA with high salt in the drinking water is a model characterized by neurohumoral activation and volume expansion. The DOCA/salt model exhibits suppression of the peripheral renin-angiotensin system but activation of the central renin-angiotensin system [86–88]. Increased activity of the sympathetic nervous system, secretion of vasopressin, and local expression of endothelin-1 are also thought to be key features in the model [86, 87]. Although this model of hypertension has been used widely in studies of the peripheral circulation [89–92], there are relatively few reports of its use to study endothelial function in the cerebral circulation. In one study, responses of the basilar artery to acetylcholine were unaffected after DOCA/salt treatment [93]. In contrast, we found that mild DOCA/salt-induced hypertension profoundly impairs cerebral microvascular endothelial function in mice [94]. Initial work to define mechanisms underlying these changes identified activation of AT1 receptors and Rho kinase as a key contributor to DOCA/salt-induced microvascular endothelial dysfunction in brain. These findings are interesting in relation to recent evidence that activity of Rho kinase is positively associated with cardiovascular events including stroke [95].
5.3 SHR/SHRSP Models of Genetic Hypertension
Endothelial function has been studied on many occasions in the cerebral circulation of SHR and SHRSP. To our knowledge, the first examination of effects of chronic hypertension on endothelial function in cerebral arterioles was performed by Mayhan et al. using SHRSP [96]. Since that initial effort, numerous reports have described impaired endothelium-dependent vasodilation in the cerebral vasculature from SHR/SHRSP compared with vessels from normotensive WKY rats [96–102]. In contrast to effects on endothelium, vascular responses to endothelium-independent agonists are typically normal in these models [97, 99, 100, 102–104]. Studies in SHRSP also indicated that impaired endothelial function in SHRSP extends beyond stimuli that are dependent on NO-signaling but also involved agents that acted on endothelium through receptor-independent mechanisms as well as agonists that act via endothelium but through non-NO-dependent pathways [99, 101, 102]. As with the Ang-II-dependent model of hypertension, the findings from SHR/SHRSP suggest that a major form of vascular dysfunction seen during chronic hypertension occurs at the level of the endothelium, while vascular muscle appears to be able to respond normally. Importantly, genetic links between impaired endothelial function and stroke have been established [18].
A number of studies have attempted to define the mechanisms that contribute to endothelial dysfunction in SHR/SHRSP with different mechanisms mediating dysfunction in different segments of the cerebral circulation (Fig. 8.2). For example, endothelial dysfunction in small cerebral arterioles can be reversed by treatment with a COX inhibitor [100]. Furthermore, inhibition of prostaglandin H2/thromboxane A2 receptors improved endothelium-dependent vasodilation [98]. In contrast, treatment of basilar arteries with a COX inhibitor does not improve endothelium-dependent vasodilation [96, 104].
Similar to what is observed in Ang-II-dependent models of hypertension, increased levels of superoxide are present in cerebral arteries from SHR and SHRSP [105]. Although there is some evidence to the contrary [106], functional studies suggest that oxidative stress contributes to endothelial dysfunction in these models. For example, an inhibitor of 20-hydroxy-5,8,11,14-eicosatetraenoic acid (20-HETE) reduced levels of superoxide and restored endothelial function to normal in middle cerebral arteries from SHR [105]. This suppression of oxidative stress may arise from inhibition of an interaction between 20-HETE and NADPH oxidase, although details for such a mechanism are limited at present. There is also evidence that the SHRSP model of hypertension is dependent on endogenously generated Ang-II. Both acute and long-term treatment of SHRSP with an ACE inhibitor restored endothelium-dependent vasodilation of cerebral arterioles, effects that are independent of changes in arterial pressure [101].
Overall, these studies highlight some of the major and complex effects of hypertension on the cerebral circulation. Different signaling mechanisms appear to play a more prominent role in causing endothelial dysfunction at different points along the cerebrovascular tree.
6 COX-Dependent Mechanisms Contribute to Vascular Dysfunction
COX is an important enzyme within the cerebral vasculature and has been linked with cerebrovascular dysfunction in several experimental models (see below). Although recent attention has focused on NADPH oxidase, COX is also an important source of ROS in the vasculature (Fig. 8.1) [58, 59] and there is evidence that COX-dependent ROS production can directly and indirectly (via ROS-dependent scavenging of NO) alter vascular tone. As discussed above, some evidence suggests that endothelial dysfunction in cerebral arterioles of SHRSP is COX-dependent (Fig. 8.2).
COX1-derived prostaglandin E2 and EP1 receptors are required for impaired endothelium-dependent vasodilation during acute hypertension induced by Ang-II infusion [8]. While it was shown that COX1 did not directly generate ROS in response to Ang-II, this COX1 signaling pathway was required for NADPH oxidase-dependent ROS production and subsequent vascular dysfunction during acute hypertension [8]. It will be of interest to determine if similar mechanisms are present during chronic hypertension.
7 Endothelium-Derived Contracting Factors
In addition to impaired endothelium-dependent vasodilator responses, hypertension also alters vascular function via the generation of EDCFs (Fig. 8.2). EDCFs may include COX-derived products, endothelin-1, and others [11]. Relatively few studies have investigated the contribution and identity of EDCFs during hypertension in the cerebral circulation. Evidence from noncerebral arteries suggests that a COX-derived product functions as an EDCF [107, 108] and studies of cerebral blood vessels agree with this concept. For example, responses of cerebral arterioles to ADP, which is an endothelium-dependent vasodilator that signals mainly through NO, are impaired in SHRSP [100]. Mechanistically, evidence suggests that this dysfunction is dependent on a COX product (Fig. 8.2) as treatment with a COX inhibitor or a thromboxane A2 receptor antagonist [98] restored endothelial microvascular responses essentially to normal [100]. Interestingly, while a key functional role is suggested for these pathways in the microcirculation, a similar EDCF does not play such a role in larger cerebral arteries [96].
8 Blood–Brain Barrier
The movement of molecules or cells out of the intravascular compartment and into the cerebrospinal fluid and/or brain parenchyma is normally highly regulated. Adjacent cerebral endothelial cells are tightly linked, forming a structural and functional barrier to the diffusion of most molecules, particularly those with poor lipid solubility [109]. This organization of endothelial cells in brain is known as the BBB (Fig. 8.3) [109–112].
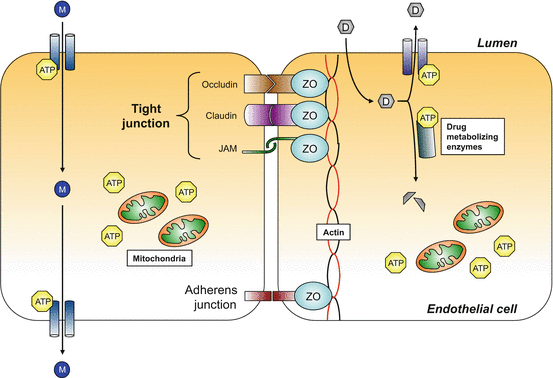
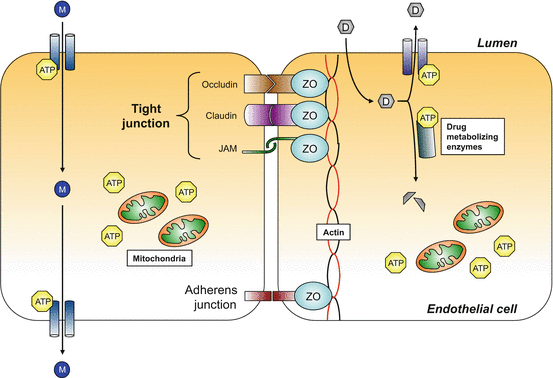
Fig. 8.3
Schematic illustration of adjacent endothelial cells that constitute the primary site of the blood–brain barrier. The physical, intercellular barrier between endothelial cells comprises the tight junctions (made up of occludins, claudins, and junctional adhesion molecule) at the apical surface and adherens junctions at the basolateral surface. These intercellular adhesion molecules are connected to the actin cytoskeleton by zonula occludens. Specialized ATP-dependent transporters facilitate the movement of molecules from the circulation into the parenchyma. Cerebral endothelial cells also contain ATP-dependent drug transporters and drug metabolizing enzymes that prevent entry of some drugs into the parenchyma. The maintenance of the BBB requires relatively large amounts of ATP and cerebral endothelial cells contain a relatively high density of mitochondria, presumably to meet this energy demand. Molecules can move across the endothelium by the activity of ATP-dependent transporters. However, some drugs can be exported out of the cells by ATP-dependent efflux pumps, or be metabolized into inactive compounds by drug metabolizing enzymes. ATP adenosine triphosphate, D drug, JAM junctional adhesion molecule, M molecule, ZO zona occludens
The main determinants of BBB permeability include: (1) the close connections between adjacent endothelial cells (mediated by tight junction proteins), (2) limited active transport and diffusion of molecules across endothelial cells, and (3) high expression of drug efflux pumps and metabolizing enzymes [113]. Although the presence of a BBB derives largely from the properties of cerebral endothelial cells, other cell types, including astrocytes and pericytes, play an important role in regulating BBB integrity by modulating endothelial function and expression of BBB components [114–117]. In the following sections we will review selected aspects of our understanding of the structure, molecular organization, and regulation of the BBB. We will then summarize concepts related to effects of acute and chronic hypertension on BBB integrity.
9 The Concept and Molecular Makeup of the BBB
Based on studies utilizing injections of dyes in experimental animals, Erlich in 1885 revealed for the first time that some blood-borne molecules cannot simply diffuse into the brain [118]. Years later, Max Lewandosky postulated that cerebral blood vessels had unique properties that allow them to block the diffusion of molecules—a function he defined as “bluthirnschranke” (blood–brain barrier in German) [109, 113, 118]. With the development of electron microscopy, Reese and Karnovsky demonstrated the fundamental role of endothelial cells in a functional BBB [113, 118, 119] showing that transport of proteins across the endothelial layer was limited by tight junctions between endothelial cells. Many subsequent studies have built upon these seminal findings, increasing our understanding of the molecular makeup of the BBB and its function in health and disease [118].
Endothelial cells of the BBB have properties that distinguish them from vascular endothelium in most other organs as well as from endothelial cells in regions of the brain where the BBB is not present (e.g., circumventricular organs). These differences can be divided into several major categories—structural, molecular, biochemical, and metabolic. Brain endothelial cells lack fenestrations and are tightly connected by interacting proteins that anchor adjacent endothelial cells while also facilitating communication between endothelial cells. These proteins are localized in structures known as tight junctions (in the apical border) and adherent junctions (near the basal border) (Fig. 8.3). Tight junctions closely connect one endothelial cell with adjacent endothelial cells. As a result, there are no structural gaps between endothelial cells in most areas of the brain.
Several families of proteins make up the structural components of tight junctions. Occludin, claudins 5, 3, and 12, and junctional adhesion molecules (JAM) 1 and 4 appear to be particularly enriched in brain endothelial cells [111]. These are integral membrane proteins that bind other tight junction proteins in the extracellular space while also interacting closely with cytoskeletal proteins. The interactions of tight junction and cytoskeletal proteins are facilitated by members of the zonula occludens (ZO) family. ZO-1 and ZO-2 proteins also facilitate regulation of endothelial and tight junction function by acting as scaffolds for signaling molecules at the cell membrane [111, 113]. Modulation of expression and the functional state (e.g., phosphorylation) of occludins, claudins, JAM, and ZO proteins is pivotal for the maintenance of BBB integrity and permeability [120]. Alterations in expression or function can lead to changes in BBB permeability and have been described in various experimental models of neurological disease, stroke, and other forms of brain injury [111, 112, 120, 121].
Endothelial cells in the brain are very metabolically active. Cerebral endothelium has a high rate of pinocytosis and expresses a series of ATP-dependent transporters (in the luminal and basolateral surfaces) that control the active transport of molecules across the cells [109, 113]. Cerebral endothelium also expresses a high level of drug efflux pumps and drug metabolizing enzymes that consume ATP and actively metabolize and transport compounds from the endothelial cell back to the blood stream [109, 113]. Brain endothelial cells characteristically contain a relatively high number of mitochondria, which presumably supports the increased energy expenditure associated with the increased expression of ATP-dependent transporters, drug efflux pumps, and metabolizing enzymes.
Although specific features can change segmentally along the vasculature, the BBB is present in vessels on the brain surface (both arterioles and venules) as well as in parenchymal vessels including capillaries [110, 122]. Capillaries may have the ‘tightest’ BBB, but the permeability of pial vessels in brain is still orders of magnitude less than similar size vessels in the peripheral circulation [122]. Other segmental differences exist in relation to the BBB. For example, the site where many interactions with circulating immune cells occur, sometimes referred to as the immunological BBB, is primarily at post-capillary venules [123], a segment of the microvasculature that can be prominently affected by hypertension (see below).
Disruption of the integrity of the BBB is a common feature of cerebrovascular and neurological disease as well as with aging [111]. Mechanisms responsible for these changes can be diverse and may include interacting oxidant- and immune-related pathways, alterations in cell surface charge, both transcellular and paracellular pathways, phosphorylation of tight junction proteins, and effects on the actin-myosin cytoskeleton [112, 124]. For example, cellular injury may lead to the increased production of matrix metalloproteinases (MMPs) and VEGF, which then degrades the integrity of tight junctions. Expression and activity of several MMPs are elevated in cerebrovascular disease including models of hypertension [121, 125–131]. For decades it has been known that hypertension induces BBB disruption [132], however the mechanisms that link hypertension with BBB disruption are still poorly understood.
9.1 Acute Hypertension
Episodes of acute hypertension can occur under both physiological and pathophysiological conditions. For example, large increases in systemic arterial pressure occur during isometric exercise and sexual activity [133, 134], however there is little indication that these activities normally injure the BBB. In contrast, large increases in arterial pressure that occur in some disease states have a major impact on cerebrovascular function and permeability of the BBB. There are many causes of acute hypertension in pathophysiology including traumatic brain injury, seizures, and severe preeclampsia and eclampsia [135]. Preexisting chronic hypertension is also a risk factor for acute hypertension [135]. BBB changes have been described in all these latter conditions. Hypertensive encephalopathy is a condition characterized by acute and severe hypertension, headache, seizures, and other neurological symptoms including cerebral edema [136]. In a model of hypertensive encephalopathy, inhibition of the delta isoform of protein kinase C prevented hypertension-induced disruption of tight junctions and increased permeability of the BBB [137]. In another study, treatment with TEMPOL (a scavenger of superoxide anion) protected against disruption of the BBB and brain edema in a model of acute hypertension, suggesting a critical role for this key oxygen-derived free radical in the process [138]. Expression of tight junction proteins (e.g., claudin 5) are reduced in response to acute hypertension [139].
Loss of BBB integrity during large acute increases in arterial pressure exhibits regional and segmental differences in the cerebral circulation [138, 140–143]. This heterogeneity likely results from a variety of factors including differences in vascular structure, intrinsic functional properties of blood vessels (autoregulatory capacity), as well as perivascular innervation by sympathetic and sensory neurons. As a result, there are regional differences in local hemodynamics and increases in microvascular pressure which are the main determinants of changes in BBB permeability under these conditions [138, 140–143]. One of the best examples of this concept is the finding that small pial venules are the major site of disruption of the BBB following acute hypertension [142, 143]. Differences in venous microvascular pressure can account for regional (and model-specific) differences that are seen in disruption of the BBB during acute hypertension [138, 140–143].
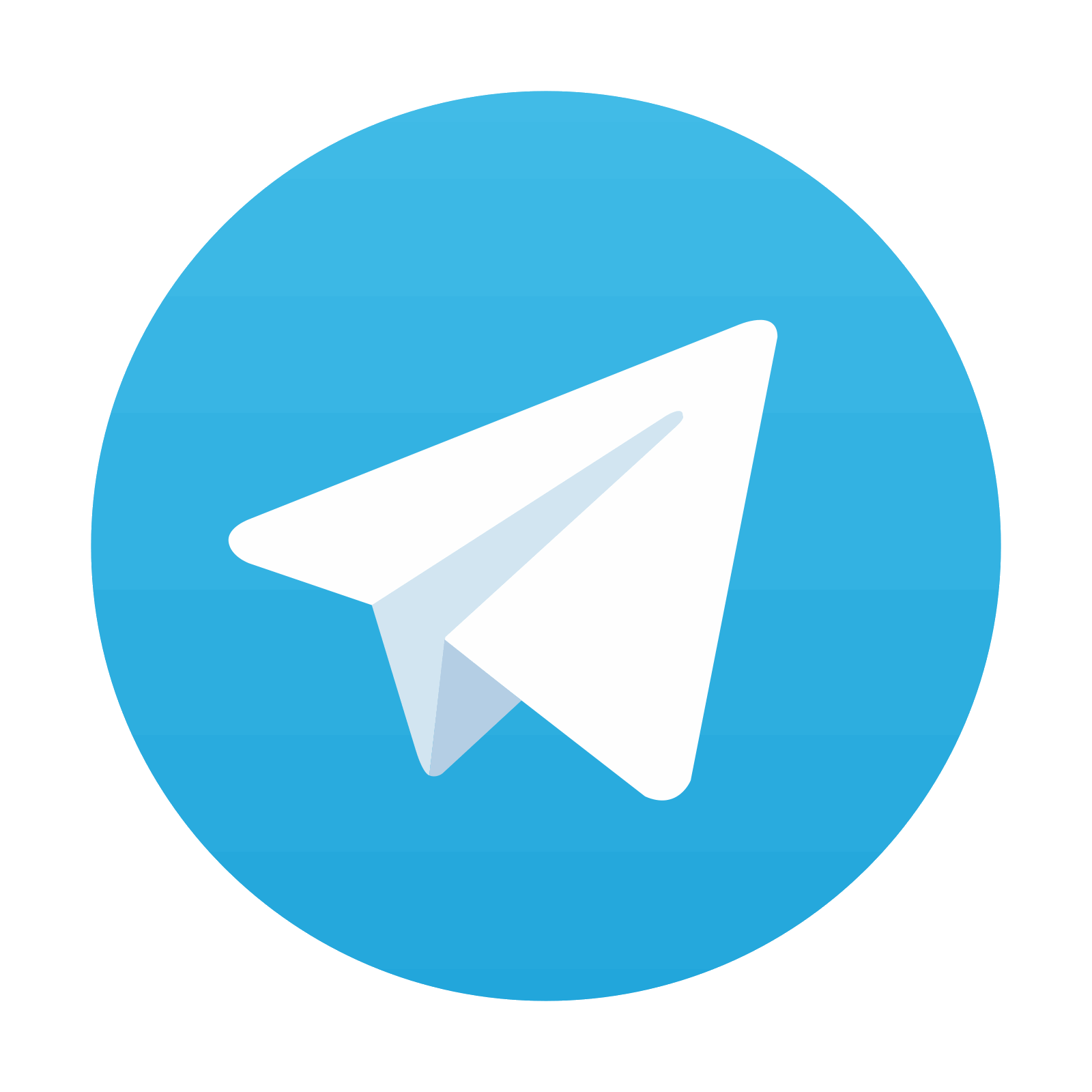
Stay updated, free articles. Join our Telegram channel
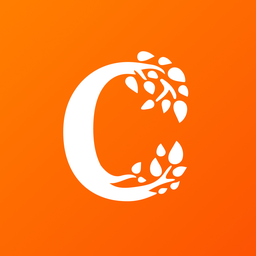
Full access? Get Clinical Tree
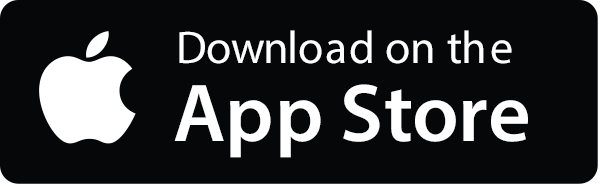
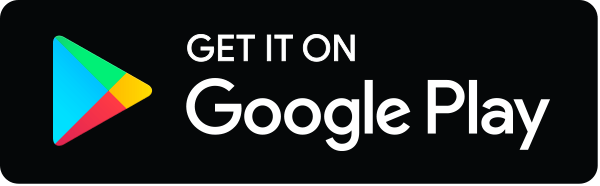