Fig. 1
Chromatin structure is a dynamic structure that contributes to gene regulation. Heterochromatin is highly compacted and usually associated with silenced genes while euchromatin corresponds to actively transcribed genes. These two chromatin states are regulated by enzymes catalyzing deposition or removal of DNA methylation or histone posttranslational modifications (acetylation or methylation for instance). Trans-acting factors, including ATP-dependent remodeling complexes are recruited by methylated DNA or histone modifications in order to translate these epigenetic marking into regulatory mechanisms. A number of noncoding RNAs, including long noncoding RNA also participate in the regulation of chromatin and subnuclear positioning contribute to the activation of repression of a number of loci
These two main chromatin states can be defined by the covalent modification of the underlying DNA, their respective level of compaction, topology, positioning, and spacing of nucleosomes, their histone code predicting how the posttranslational modifications of specific amino acids of the core histones (H2A, H2B, H3, and H4) are translated into distinct information [4], the presence of histone variants, composition in non-histone binding factors, the spatial localization within the nucleoplasm and its dynamics during cell cycle (Fig. 1). In higher eukaryotes, heterochromatin is enriched in methylated DNA, hypoacetylated histones, lacks H3K4 methylation but is enriched in histone H3K9 dimethylation and trimethylation or H3K27 and H4K20 methylation at the level of histones and binds HP1 [5, 6]. On the other hand, euchromatin is less methylated at the DNA level, hyperacetylated and enriched in H3K4 methylated residues (Fig. 2).


Fig. 2
Sites of posttranslational modifications on the histone tails. Modifications located in their amino (N)-terminal tails of the four core histone are represented. Only acetylation (green) and methylation (red) of lysine residues is indicated. Several types of covalent modifications are reversible and some histones can be either methylated or acetylated. The respective role in either gene activation (Go) or repression (STOP) is indicated
The identity and transmission of these chromatin states are controlled and regulated by processes involved in controlling many cellular functions such as gene expression, genomic stability, X inactivation, genomic imprinting, gene silencing, etc., globally referred to as “Epigenetics.” This word derives from the word “epigenesis,” an ancient theory stating that human being gradually develops from an undifferentiated mass after different steps to become more complex. It is opposed to the theory of pre-formationism that considers that the embryo appears as a homunculus in the male germ cell and develops once in the female. The term “epigenetics” was proposed in middle of the twentieth century by Waddington to describe the development of a phenotype based on a genetic potential subjected to influence of its environment [7]. Currently, epigenetics defines reversible and dynamic modification of gene expression processes that affect gene expression without affecting the genetic code and is transmitted through cell divisions and meiosis without change in the nucleotide sequence. A related term, epigenomics refers to the global epigenetic profile of a given cell (or organism) at a given time. Based on this definition, each individual carries a unique epigenome even in the case of monozygotic twins, a phenomenon referred to as “epigenetic drift,” reflecting the lifetime environmental influence on the epigenetic status [8, 9]. Epigenetic changes are governed by the presence or absence of chemical residues decorating the DNA sequence of the chromatin fiber but also involve a number of noncoding RNAs including micro RNAs or LncRNAs (long noncoding RNAs).
In pathologies, many epigenetic alterations have been described. The goal of this chapter is not to provide a detailed review of all the epigenetic processes described so far but rather to give an overview of the main features of these epigenetic changes, especially during differentiation of the central nervous system and to give a few examples of the involvement of epigenetic alterations in human pathologies.
2 Epigenetic Processes in Mammals
2.1 DNA Methylation
In vertebrates, CpGs dinucleotides are underrepresented (one fifth of the expected frequency) and in 60–90 % of cases, these dinucleotides are methylated on the carbon 5 of the cytosine residue (m5C) [10, 11]. Cytosine is the main chemically modified base in higher eukaryotes [12].
Cytosine methylation protects DNA from enzymatic digestion by restriction enzymes and initial global digestion pattern analysis combined with the recent mapping of methylated CpGs across the murine and human whole genomes revealed that CpG poor regions, including exons, introns, intergenic regions, and repeated sequences, are highly methylated (from 80 to 90 % are CpG methylated). However, a very few CpGs-rich regions appeared sensitive to the action of these enzymes suggesting that some regions of the genome contain unmethylated CpGs [13]. These small regions of 0.5–2 kb, called CpG islands represent approximately 1 % of the genome and are characterized by a G + C content above 50 % and a CpG/GpC ratio close to 1 [14, 15]. CpG islands are frequently found upstream of constitutively expressed genes [16] or in 40 % of tissue-specific genes [14].
In a given genome, the methylation patterns are precisely established with a lack of methylation of CpGs islands and a more general methylation of other dispersed CpG sequences. A few exceptions of hypermethylated CpG islands have been described in the case of parental imprinting and dosage compensation of one of the two X chromosome [17, 18]. Unlike the DNA sequences, methylation profile is not inherited from the gametes. Almost all methylation is erased in the early embryo and a new pattern is established at the time of implantation. Furthermore, this uneven bimodal distribution of methylated CGs depends on the type of tissue, with brain or oocytes harboring a very low level of methylation [19–22]. Methylation of promoter regions is usually associated with gene silencing but beside regulation of transcription, splicing is also influenced by DNA methylation [23] possibly through the binding of proteins with a high affinity for methylated DNA, which would regulate the binding of splicing factors [24]. The intragenic DNA methylation promotes transcriptional elongation by inhibiting transcription from alternative promoters contained within genes [25, 26] and methylation regulates alternative promoter usage, short and long noncoding RNAs production, alternative RNA processing, as well as enhancer activity. Furthermore, a very high proportion of methylated CpG is found at transposable elements and contributes to transcriptional repression putting DNA methylation in the center of the multiple pathways involved in genome regulation.
2.1.1 Enzymes Involved in DNA Methylation
The DNA methyltransferases catalyze DNA methylation. Four members of this family have been identified, each playing a specific role: DNMT1, DNMT3A, DNMT3B, and DNMT3L. DNMT1 has a high affinity for hemi-methylated DNA and is mainly involved in the maintenance of DNA methylation after each round of replication [27–29].
De novo methylation is catalyzed by DNMT3A and DNMT3B [30]. DNMT3A is present in differentiated cells. Dnmt3a −/− mice do not exhibit embryonic lethality but die after about 4 weeks of life [30]. This enzyme is necessary for the establishment of parental imprinting in germ cells [31, 32]. DNMT3B is abundantly expressed during the early stages of embryogenesis and in tumoral tissues [33]. This enzyme is primarily responsible for the methylation occurring after embryonic implantation [34] and Dnmt3b −/− mice die during embryonic development [30]. In adult tissues, DNMT3B is mainly found in the testes, bone marrow, and thyroid [33]. This enzyme catalyzes methylation of centromeric satellites repeats [30], explaining the centromeric instability observed in patients affected with the ICF syndrome (Immunodeficiency, Centromeric instability, and Facial abnormalities) [35].
Another member of this family, DNMT3L, does not contain the catalytic site present in the other DNA methyltransferases. This enzyme is highly expressed in germ cells where it exerts its function. Dnmt3l −/− mice are viable and develop normally. However, DNA methylation of male and female germ cells is altered, leading secondarily to male sterility [32, 36, 37]. DNMT3L acts as a cofactor for the other DNMT3, stimulating their activity [38, 39].
2.1.2 Removal of Methylation and Hydroxymethylation
Given the key role of DNA methylation in developmental processes, a certain plasticity in the deposition but also removal of this epigenetic mark is required. Early studies demonstrate that demethylation is an active event and does not occur passively in the absence of remethylation during DNA replication. However, for many years, the mechanisms of active demethylation have been controversial [40, 41] until recently with the identification of the TET proteins. So far, three TET proteins, named TET1 to TET3 have been identified and implicated in the replication-independent DNA demethylation pathway [41, 42]. These proteins are 2-oxo-glutarate Fe2+-dependent dioxygenases, which convert 5mC to 5hmC using α-keto-glutarate as a co-substrate [43, 44]. One possibility is that 5hmC is further oxidized to 5-formylcytosine (5fC) or 5-carboxylcytosine (5caC) which can be removed by glycosylation enzymes [45, 46]. Alternatively, 5hmC, which is not recognized by the maintenance DNA methyltransferases, DNMT1, could be diluted at each subsequent round of replication.
Other enzymes such as APOBEC or AID deaminase induce active demethylation after deamination of 5mC residues especially in the brain [42, 47]. These modified bases are then removed by glycosylases, which generate apyrimidic acid subsequently removed by base excision repair.
Among the different TET enzymes, TET2/3 are the most highly expressed in the cortex [48, 49] and might be involved in the activation of genes involved in neuronal differentiation. Unlike 5mC, which is usually found in heterochromatin, 5hmC, now considered as the sixth base of DNA is highly abundant in the brain and associated with euchromatin and regions permissive for transcription in both neurons and ESCs [49–51]. In neurons and neuronal progenitors, 5hmC is enriched in tissue-specific genes and 5hmC levels associated with these brain-specific genes increase during early differentiation [49]. Increase in 5hmC during early differentiation of NPCs to neurons is associated with higher transcriptional activity especially in genes involved in neuronal differentiation, migration, and axon guidance [49].
2.2 From Methylated DNA to Chromatin Fiber
Eukaryotic DNA is tightly packed around histone octamers to form nucleosomes. Nucleosomes must be packed to achieve the 10,000–20,000-fold compaction necessary to fit a genome into a nucleus of a few microns. Beside DNA methylation, compaction of the chromatin fiber also depends on changes at the level of nucleosome. A few examples of factors or modifications involved in nucleosome compaction but also in transcription, replication, and repair are developed below.
2.2.1 Proteins with Affinity for Methylated DNA
Methylation may control the expression of genes by two types of mechanisms, either directly, by preventing the access of transcription factors to their(s) target sequence(s), or indirectly through binding of proteins with affinity for methylated DNA. Many transcription factors contain CpG dinucleotides in their binding sequence [52] and their affinity is decreased when these sequences are methylated [53, 54]. This aspect is not further developed here.
The identification in the 1990s of a family of proteins having a high affinity for the fifth base of the DNA, revealed the existence of specific factors able to bind methylated DNA. This family includes five members (MeCP2, MBD1, MBD2, and MBD3) characterized by the presence of a domain called MBD for “Methyl CpG Binding Domain” binding methylated CpG dinucleotides [55, 56]. Except MBD3, which contains an amino acid substitution that prevent binding to methylated DNA, the four other members of this family may participate in the control of gene expression thanks to the presence of a transcriptional repression domain (TRD). The fifth member, MBD4 has an N-Glycosylase activity and participates in DNA repair [57], but a recent report also suggests a role as transcriptional repressor [58].
These methyl-binding proteins recruit specific factors involved in transcriptional repression or heterochromatin formation. MeCP2 interacts with the transcriptional repressor Sin3A [59, 60] and a component of the SWI/SNF complex, Bhrama [61] but may also exist as a monomer, in particularly in the brain [62]. MBD2 and MBD3 are compounds of the Mi-2/NURD remodeling complexes [56, 63], and MBD1 recruits the histone methyltransferase, SETDB1 and participates in the formation of heterochromatin [64, 65].
Two other families of proteins having affinity for methylated DNA have also been identified more recently: Kaiso, ZBTB38, and ZBTB4 proteins belonging to the zinc finger proteins family and acting as transcriptional repressors [66] and a group of proteins containing a SET and RING finger associated domain and ubiquitin-like motif, UHRF1 and UHRF2 with a strong affinity for hemimethylated DNA. These proteins colocalize with DNMT1 during the S phase and could also participate in the maintenance of methylation patterns during replication [66].
The particular state of methylation of certain CpG islands could involve the CXXC domain proteins able to bind to unmethylated CpG-rich DNA. A member of this family, CFP1 (CXXC Finger Protein 1), a member of the SET1 complex involved in the trimethylation of lysine 4 of histone H3, binds unmethylated CpG islands and promotes H3K4 methylation [67]. As H3K4 methylation has been proposed as an inhibitor of DNMTs recruitment, one might think that H3K4 methylation-dependent CFP1 protects CpG islands methylation. However, depletion in CFP1 is not sufficient to increase CpG island methylation [68–70].
2.2.2 Histone Modifications
Beside DNA methylation, regulation of chromatin compaction and transcription also depends on changes in the nucleosomes catalyzed by histone modifiers acting as “writers” able to introduce covalent modifications to histone residues. Over 100 distinct histone modifications with intricate functions in providing signals that regulate chromatin dynamics, transcription, replication, repair, or genome stability have been described. These modifications include methylation, acetylation, phosphorylation, ubiquitination, sumoylation, ADP-ribosylation, deimination, citrullination, or proline isomerization [71]. Histone modifications are reversible and several enzymes acting as “erasers” have also been identified. Furthermore, as described for DNA methylation, histone modification can also modify the accessibility of chromatin to the machinery involved in the regulation of different DNA transactions but can also serve as a platform for the recruitment of readers or effectors of this epigenetic code. Among the different posttranslational modifications of histones, we more specifically focus on the role and regulation of acetylation and methylation of lysine involved in activation or repression of transcription (Fig. 2).
Histone acetylation was the first posttranslational histone modification described [72]. Acetylation of lysine neutralizes the positive charge of these amino acid residues, weakening thereby the charge-dependent interactions between a histone and nucleosomal DNA, linker DNA or adjacent histones. Hyperacetylated histones are usually associated with actively transcribed genes since the cumulative charge neutralization by multiple lysine acetylations increases DNA accessibility and facilitates transcription [73–75]. In addition, histone acetylation modulates the firing of replication origins and accessibility of DNA to replication factors [76, 77] or facilitates DNA access for repair factors in the context of DNA double strand breaks [78].
Histone acetylation is highly dynamic. Lysine residues are acetylated by lysine acetyltransferases (HATs) with low substrate affinity. Acetyl-lysines are recognized by proteins containing a bromodomain present in a wide range of chromatin-associated proteins. Histone deacetylases (HDACs) are enzymes that balance the acetylation levels on chromatin and play essential roles in regulating gene transcription. Acetylation of nucleosomes and gene bodies facilitate efficient polymerase transit by loosening histone–DNA contacts with subsequent deacetylation tightening histone-DNA contact and chromatin reassembly after transcription.
Histone lysines are methylated to form monomethylation, dimethylation, and trimethylation on lysine at position 4, 9, 27, and 36 of the amino-terminal tail of histone H3 (H3K4, H3K9, H3K27, H3K36), lysine 79 of the globular domain of H3 (H3K79) or lysine at position 20 of histone H4 (H4K20) (Fig. 2). A distinct pattern of histone methylation is found at promoters, insulators, enhancers, transcribed regions, or repetitive DNA sequences. Monomethylation of H3K4, H3K9, H3K27, and H4K20 and trimethylation of H3K36 are usually observed at transcribed regions while trimethylation of H3K9, H3K27, and H4K20 are associated with repressed genes and heterochromatin regions.
Methylated lysines are bound by different protein motifs including Tudor, Chromo, PWWP, MBT, and PHD domains [79]. In addition, by enhancing the affinity of certain promoters for histone tails, histone methylation also enhances nucleosome stability.
2.3 Organization of Chromosomes in Interphase Nucleus
In interphase nuclei, it was first supposed that chromosomes would entangle after decondensation. However, at the end of the eighteenth century, Rabl and Boveri demonstrated that plant chromosomes conserve polarity in interphase nuclei and hypothesized that chromosomes occupy discrete territories within the nucleus. Moreover, initial studies of the β-globin loci in humans and mice have shown that active promoters, enhancers, and distant regulatory elements located on linear DNA regions, at a distance of tens of kilobases are co-localized in the interphase nucleus forming a central regulatory structure associated with RNA polymerase II called “chromatin hub” [80, 81]. A clear demonstration for the existence of chromosome territories (CTs) came approximately three decades ago with the work of Cremer and collaborators who observed that UV laser irradiation of discrete regions of Chinese hamster cell nuclei damages only a small subset of mitotic chromosomes [82]. Furthermore, distinct territories do not overlap and homologous chromosomes generally occupy non-adjacent territories [83].
Based on these observations and on publications highlighting the importance of nuclear positioning, we now know that the organization of the genome within the eukaryotic nucleus is not random and dynamic and that the spatial distribution of DNA sequences controls nuclear processes.
Gene-poor CTs are usually found at the nuclear periphery whereas gene-rich chromosomes localize internally [84, 85]. This spatial organization of CTs based on gene-density is conserved in primates [86], whereas the idea that chromosome size influences localization remains conflicting and partly unresolved [83, 85, 87]. Consequently, the plasticity of the chromatin fiber is limited by interactions and higher order-structures, which may be tailored by transcriptional activity. Thus, differentiation processes, requiring the sequential expression of specific genes, may participate in the shaping of chromosomal domains depending on cell-type specific transcriptional competence.
The nuclear periphery is generally associated with heterochromatin and silent chromatin modifications while the repositioning of genes away from the nuclear periphery is usually associated with relocalization of loci away from the nuclear envelope. For instance, upon differentiation of mouse ES cells into neural progenitors, the Mash1 locus is displaced away from the nuclear envelope concomitantly with Mash1 gene activation, while expression of some neighboring genes is not affected [88]. This displacement correlates with histone modifications principally at the Mash1 gene. Interestingly, ES cells mutated for histone and DNA methyltransferases (HMTs and DNMTs, respectively) do not exhibit changes in the association of the locus to the nuclear periphery and it was suggested that histone acetylation rather than methylation could be functionally linked to the control of chromatin association to the nuclear rim indicating a link between epigenetic changes and subnuclear distribution of functional domains.
2.4 Implication of Long Noncoding RNA in Chromatin Regulation
A large proportion of the genome is transcribed into noncoding RNAs, which participate in the regulation of gene expression. Only the example of long noncoding RNAs is developed here. Long noncoding RNAs (or LncRNA) are heterogeneous group of noncoding transcripts with a size of 200 nucleotides or more [89]. These RNAs transcribed by RNA polymerase II are the most common noncoding RNAs in mammals and are predominantly found in the nucleus. LncRNAs are transcribed from intergenic regions, in antisense, overlapping, intronic and bidirectional orientations relative to protein-coding genes or from regulatory regions such as UTR, promoters or enhancers. Their expression is tightly regulated and, as observed for protein-coding genes, regions encoding LncRNAs are enriched in H3K4 trimethylation at promoters and H3K36 is associated with LncRNAs elongation [90]. The LncRNA have a 5-methyl-guanosine 5′ cap and are often spliced and polyadenylated but devoid of evident open reading frame. Their intrinsic nucleic acid nature confers to LncRNAs the capacity to bind proteins and to mediate base pairing interactions as described for other noncoding RNA such as microRNAs, small nucleolar RNAs, or small nuclear ribonucleoprotein particles. Expression of LncRNAs seems to be tightly regulated with a high tissue- and differentiation-dependent specificity.
Most of the nuclear LncRNA have been characterized for their role as epigenetic modulator at specific loci [89, 91–94] and thanks to their flexible and modular scaffold, they form higher order structures that facilitates interaction with proteins (reviewed in [95]). By recruiting DNMT3, the polycomb repressive complex (PRC2) [89, 96] or the H3K9 methyltransferase [97, 98], they contribute to the formation of heterochromatin and gene repression. The first and best-characterized examples of LncRNAs are those involved in X inactivation and parental imprinting (for review, [95, 99, 100]).
3 Chromatin Regulation and Neural Development
During development, the epigenome undergoes cycles of demethylation and methylation enabling the setting of cell or tissue-specific patterns. DNA methylation and epigenetic changes play key role in neural development, plasticity, learning and memory. Recent literature has shown that the stemness of neuronal stem cells is maintained by the epigenetic suppression of neuronal and glial cells, whereas NSCs differentiation requires the removal of repressive epigenetic marks at genes involved in glial or neuronal fate specification making each neuronal subtype (neurogenic phase). During all stages of neural development, from the fate switches of neural precursor/progenitor cells to activity-dependent synapse maturation, chromatin modifications are important regulators of the gene expression that control developmental programs. Furthermore, accumulating evidence showed that in the mammalian brain, DNA methylation and chromatin modifications play pivotal role in coordinating developmental programs while alteration in a certain number of epigenetic factors are associated with neuropsychiatric disorders. A few examples are developed below.
The expression of DNA methyltransferases has been determined in the central nervous system [101–103]. Interestingly, non-dividing neurons still hold substantial level of these enzymes indicating a key role for DNA methylation in non-dividing neuronal cells suggesting that these enzymes are not only required for the maintenance of DNA methylation pattern after each cycle of replication but also contribute actively to the regulation of gene expression and differentiation. Conditional invalidation of either Dnmt1 or 3b in the nervous system revealed a role for these enzymes in neural cell fate specification, neuronal maturation, synapse formation and cell survival. During embryogenesis, Dnmt1 is expressed at high level and remain substantially high in the adult brain in the mouse. DNMT1 is also strongly expressed in post-mitotic neurons and plays important roles in neuronal differentiation, migration, and central neural connection [104]. Dnmt3b is mainly expressed at early embryonic stages and neural progenitors before E15.5 whereas Dnmt3a is mainly expressed in later embryonic stages and in adult neural precursor cells, maturing neurons, oligodendrocytes and astrocytes [101]. Furthermore, in agreement with a role for DNA methylation in the CNS, changes in expression of the different enzymes catalyzing DNA methylation have been described in a number of diseases such as schizophrenia [105], bipolar disorder [106], suicidal/major depression disorder [107], or epilepsy [108].
Another example showing the importance of DNA methylation and chromatin changes in brain function comes from Mbd1-mutant mice [108] since adult Mbd1 mutants show impaired spatial learning and reduction in long-term potentiation of the hippocampus.
Neuronal development and cognitive functions have also been correlated with the function of histone modifying enzymes. Mammals have 18 HDACs grouped into four groups, but their role in NSCs and neuronal development is not fully defined.
Class I HDACs (HDAC 1, 2, 3, 4) are localized in the nucleus. HDAC1 is enriched in the glia in the adult brain. HDAC2 is upregulated in NSC and in the mouse, overexpression of hdac2 in the hippocampus regulates synaptic plasticity, long-term potentiation, and memory formation [109]. HDAC2 overexpression has been observed in Alzheimer’s disease along with genes implicated in learning and memory [110].
Class II HDACs (4–7, 9, and 10) are able to shuttle in and out of the nucleus and are upregulated in differentiating NSCs [111] and HDAC5 regulates NSC neuronal differentiation [112]. HDAC4 and 5 are involved in neuronal maturation and neuroprotection and are enriched in the brain [113].
In the mouse, increased histone acetylation by HDAC inhibitors induces learning and memory recovery [114–117] while Dnmt inhibitors prevent memory formation [118–120]. Furthermore, mutations in CREB-binding protein (CBP), a transcriptional regulator with acetyltransferase activity are involved in the cognitive disturbance in the Rubinstein–Taybi syndrome [109] suggesting a key role for histone acetylation in neuronal plasticity.
Histone methylation is also emerging as a central pathway in brain development and function and maintenance of a bivalent chromatin state enriched for both H3K4me3 and H3K27me3 are essential for proper neurogenesis by maintaining developmental genes in a poised state upon lineage commitment.
The polycomb group (PcG) and trithorax group (trxG) gene products, respectively repress or activate transcription and control the specification and maintenance of cell identity by modifying chromatin structure. In embryonic brain, both PRC1 and PRC2 complexes are required in the transition from neurogenesis to gliogenesis [121, 122]. The PRC2 core component EZH2 is essential for stem cell maintenance and fate specification. Ezh2 is highly expressed in proliferating cells and its expression declines in differentiated neurons [123]. In adults, polycomb-mediated gene silencing is required for the maintenance of the NSC population.
MLL family members can physically interact with and recruit H3K27-specific demethylases UTX and JMDJ3 to specific neurogenic loci implicated in neuronal differentiation. The MLL1 (Mixed Lineage Leukemia-1) histone H3K4 methyltransferase, a member of the trxG complex is required for adult neuronal differentiation but not for glial or oligodendrocyte differentiation [124], controls the Dlx2 complex and is associated with fear memory [125].
Postnatal neuron-specific deletion of the G9a/GLP histone methyltransferase elicits deregulation of H3K9 methylation and cognitive and behavioral defects [126].
Overall, neurogenesis is regulated by combination of methylation changes and histone modifications forming an intricate epigenetics network. General neurogenesis is initiated by activation of Neurogenin, which by forming a complex with SMAD and p300 stimulate histone acetylation and activate neuronal specific genes such as NeuroD [127]. Concomitantly, hypermethylation of the glial fibrillary acidic protein gene (GFAP) facilitates MeCP2 recruitment and formation of a repressive complex involving Sin3 and HDAC1, which represses the glial phenotype [128]. An additional epigenetic checkpoint in neuronal differentiation involves SMRT which is essential for repression of the H3 trimethyl K27 demethylase, Jumonji domain-containing 3 (jmjd3) [129].
Many LncRNAs are involved in proliferation, differentiation, and maintenance of neural cells at different levels. They are expressed in precise regional and temporal patterns in the developing neural system and adult brain. Spatiotemporal expression profiles of highly conserved orthologous LncRNA display remarkable similarities in the brain suggesting a key role during evolution. For example, the DLX6 antisense LncRNA 1 (Evf2) is transcribed from an enhancer that affects forebrain development by modulating expression of the distal-less homeobox 5/6 transcription factors [130]. The SOX2OT (Sex-determining region Y-box 2 distal overlapping transcript) are LncRNAs transcribed from the same genomic locus as SOX2 implicated in the differentiation of neuronal precursors.
In addition, several ncRNAs mediate epigenetic mechanisms in neural processes. Overall, LncRNAs confer neural cells the capacity to exert precise spatiotemporal control genes involved in neurobiological complexes and defects in LncRNAs can lead to a number of diseases (for review [131]) but the precise role of many of these LncRNAs in brain development and adult neurogenesis is still unclear.
4 Epigenetics and Neurological Diseases
Abnormal methylation patterns or defects in proteins associated with methylated DNA have been identified in various genetic diseases such as the ICF syndrome (Immunodeficiency, Centromere instability and Facial abnormalities) [134, 135], the Rett syndrome or other mental retardation syndromes [135, 136]. In addition, changes in methylation of certain loci subject to parental imprinting affect cognition, learning, social adaptation, and stress resistance [137, 138], but could also be involved in neuropsychiatric diseases such as autism, schizophrenia, depression and Alzheimer’s disease [139, 140].
Also, in agreement with a key role for epigenetic in neurological diseases, individuals with mutations in genes encoding other proteins that regulate chromatin structure develop mental retardation syndromes. For instance, constitutive mutation in the SWI/SNF DNA helicase ATRX are involved in the ATR-X syndrome characterized by severe mental retardation, microcephaly, seizure, genital abnormalities, growth retardation, and alpha-thalassemia [141]. The gene encoding the SMC1/JARID1C histone H3K4 demethylase is mutated in the Claes–Jensen X-linked mental retardation syndrome. A few additional examples illustrating the different levels of chromatin regulation mentioned in the second paragraph are developed below.
4.1 Imprinted Defects, Mental Retardation and Autistic Behavior
Most of the autosomal genes are expressed from both alleles. However, a certain number of genes are expressed from only one allele per genome, depending on their parental origin. This phenomenon named “parental imprinting” involves the deposition of different epigenetic marks involved in the designation of the parental origin and the maintenance of this parent-of-origin designation during development and differentiation. Approximately 100 imprinted genes have been identified in mammals so far (http://har.mrc.ac.uk/research/genomic_imprinting; http://igc.otago.ac.nz/home.html).
DNA methylation plays a key role in genomic imprinting. Most of the imprinted genes are organized in loci spanning 1–3 Mb in length containing biallelically expressed genes interspersed with paternally expressed genes and maternally expressed genes and long noncoding RNAs. Imprinted loci are regulated by CpG-rich cis-acting regulatory elements named Imprinting Control Regions (ICR) of a few kilobases in length and regulated by differential DNA methylation (Differentially Methylated Region, DMR) depending on the parental origin. The ICR methylation pattern is established in the gametes by DNMT3A and DNMT3L [31, 37, 142] and maintained during early embryogenesis by DNMT1 [143, 144]. Marks inherited from the parent are erased in the primordial germ cells of the offspring and then restored according to the sex of the embryo [144]. Beside imprinting loci, a few isolated imprinted genes have also been described and are usually regulated by differential methylation and posttranslational modification of histones.
Most of the imprinted genes are implicated in prenatal development, in particular in the brain where imprinted genes are involved in modulating metabolic axes, behavior, learning, and maternal care.
A number of imprinting disorders have been described including transient neonatal diabetes [145], maternal and paternal uniparental disomy of chromosome 14 [146], growth disorders such as Beckwitt–Wiedeman and Silver–Russell syndromes [147–149] linked to the 11p15 locus or pseudoparathyroidism 1b linked to the 20q locus.
Prader–Willi syndrome (PWS) and Angelman syndrome (AS) are clinically distinct complex disorders linked to chromosome 15q11-q13. They are both characterized by neurological, developmental, and behavioral phenotypes. Both disorders can result from microdeletion, uniparental disomy, or defect in the 15q11-q13 imprinting center. The abnormality is carried by the paternal chromosome for PWS or the maternal allele for AS. PWS is characterized by neonatal hypotonia, childhood obesity, hypogonadism, cognitive impairment, and behavioral manifestation including obsessive-compulsive symptoms and hypothalamic insufficiency. This syndrome is linked to de novo paternal 15q11-q13 deletion in 70 % of cases, maternal disomy in 29 %, and methylation defect in 1 %. AS is characterized by mental retardation, speech impairment, ataxia, seizure, and microcephaly. In 70 % of cases, the disease is caused by deletion of the 15q11.2-q13 locus, paternal disomy in 7 % of cases, methylation defect (3 %), or epimutation in rare cases.
Interestingly, the 15q11.2-13.3 contains numerous genes with critical neurological functions such as CHRNA7 (cholinergic receptor nicotinic alpha 7), UBE3A and GABRB3 and deletions and duplications of the locus are observed in 1–3 % of patients with autism suggesting a key role for this locus in the neuronal development [150]. The main cause of AS is the loss of function in the brain of the UBE3A gene which codes for the E6-AP protein implicated in the transfer of small ubiquitin molecules to certain target proteins, to enable their degradation and might regulate synapse function through protein degradation pathways. The pathogenic mechanisms that underlie autism and AS due to the excess or lack of UBE3A are still unknown. These phenotypes could be caused by the misregulation of UBE3A targets influencing spine density, synapse, and neuronal function.
Beside protein-coding genes, several noncoding RNAs are also present in the PWS/AS region including the neuron specific SNRPN long noncoding RNA and a cluster of small nucleolar RNA transcripts (sno-RNAs) encompassing the UBE3A–AS region. In addition, the imprinting control region is bound by MeCP2, which is mutated in the Rett mental retardation-syndrome (OMIM 312750; [151, 152]). By binding to the PWS-ICR, MeCP2 has a role in loop formation acting as a neuronal chromatin structural organizer during neuronal maturation. Among the genes regulated by formation of these higher-order structures at the 15q11.2-13.3 locus, MeCP2 might positively regulate the expression of the CHRNA7 gene which is significantly reduced in Rett syndrome and frontal brain in autistic patients [140, 153, 154].
Overall, these results suggest overlapping epigenetic pathways between locus-specific abnormalities and autism.
4.2 Disorders of Unstable Repeat Expansions
Repeat expansion diseases are caused by an increase in the number of trinucleotide repeats and share some common features. These short repeats are polymorphic in the general population. Beyond a certain number of repeats, they become unstable and tend to increase at the next generation (dynamic mutation). The disease appears when the number of triplets exceeds a certain critical threshold. Trinucleotide repeats are either found in coding region resulting in the production of a protein with altered function (i.e., Huntington disease), or in noncoding regions resulting in an altered transcription and loss of protein synthesis (Fragile-X syndrome, Friedreich’s ataxia, myotonic dystrophy) [155], and pathophysiology of these diseases implicate epigenetic changes and heterochromatin formation [156].
Fragile X syndrome (FXS) (MIM # 300624) is the most common form of inherited mental retardation with a prevalence of 1/2,500 (Orphanet Database), characterized by a delayed language, autistic-like behavior and sometimes dysmorphic signs.
FXS is caused by expansion of a CGG trinucleotide in the 5′ UTR of the FMR1 gene located on Xq27.3. In the normal population, the number of repeats varies from 6 to 54. Between 55 and 200 CGG, the repeats become meiotically unstable (premutation) especially in the maternal germ line while the full mutation corresponds to more than 200 repeats and is accompanied by a hypermethylation of the repeats and neighboring sequences [157, 158]. FMR1 hypermethylation could act directly by preventing the binding of transcription factors. However, features of inactive chromatin have also been observed on mutated alleles like lysine hypoacetylation of histone H3 and H4 and H3K9 methylation [159, 160] suggesting that expansion of methyl-sensitive repeats contributes to the phenotype. These changes in the chromatin structure lead to the silencing of the FMR1 gene and absence of the FMRP protein, a regulator of translation in brain neurons [161, 162]. FMRP binds approximately 4 % of the mRNA produced in the brain and may be involved in the nuclear export and targeting of certain transcripts to the ribosome [163]. In the synapse, FMRP plays a role in regulating translation of proteins involved in certain pathways including the mGluR-LTD receptor (metabotropic glutamate receptor long term depression-dependent) [164]. Its loss of function would explain the cognitive deficits found in the fragile X syndrome.
Another interesting disease linked to triplet expansion is the Friedreich’s ataxia (FRDA) (MIM # 2293000), an autosomal recessive neurodegenerative disease that begins in childhood and is characterized by difficulties to coordinate movements, dysarthria, loss of reflexes, pes cavus, scoliosis, cardiomyopathy, and diabetes mellitus. The causative gene, FXN, encodes the Frataxin, a protein involved in the assembly and transport of iron-sulfur proteins of the mitochondrial respiratory chain. FRDA is caused by the expansion of a GAA trinucleotide in the first intron of FXN. Repeats range from 6 to 34 in the general population and are over 66 in patients. Expansion is associated with a decrease in FXN transcription level and different mechanisms could be implicated in gene silencing [165].
First, it has been shown that GAA repeats adopt an unusual conformation able to inhibit transcription in vitro [166]. Then, a role for heterochromatin formation has been proposed based on the observation of patient’s samples [167, 168]. Moreover, in FRDA patients specific CpG sites are hypermethylated in the FXN intron 1 compared to control and hypoacetylation of histones H3 and H4, hypermethylation of H3K9 are thought to modulate FXN promoter activity [167, 168].
Moreover, in favor of a role for chromatin remodeling and heterochromatinization in the pathophysiology of these two examples of triplet expansion diseases, reactivation of FMR1 and FXN transcription are induced by DNA hypomethylating (5-aza-2′-deoxycytidine) or histone hyperacetylating agents [169, 170].
4.3 Hereditary Sensory Neuropathy with Dementia and Hearing Loss Type 1
Hereditary sensory neuropathy type 1 (HSAN1) is an autosomal dominant neurodegenerative pathology that clinically manifests as loss of sensation, dementia, and hearing loss (MIM # 614116). Affected individuals have early mortality and often require total care because of dementia, loss of ambulation from predominant sensory ataxia, and hearing loss [171, 172]. Genome-wide linkage analysis of several kindred with HSAN1 symptoms led to the identification of mutations in the gene encoding the DNA methyltransferase 1 (DNMT1) on 19p13.2 as the cause of the pathology. The DNMT1 protein contains a C-terminal catalytic domain and an N-terminal regulatory region involved in protein–protein interactions and required for the activation of the catalytic activity carried by the C-terminal region [173]. The identified mutation occurs in the targeting-sequence domain that regulates DNMT1 binding to chromatin during the late S phase and is responsible for the persistent association during the G2 and M phases. During replication DNMT1 is associated with the replication fork and remethylate the neosynthesized DNA strand. Heterochromatin is replicated at the end of the S-phase and persistent association of DNMT1 after the S phase could be required for the proper remethylation of this heavily methylated part of the DNA. The mutations in HSAN1 impair DNMT1 targeting to heterochromatin in G2 but the direct link between DNMT1 mutation and the HSAN1 phenotype requires further investigation together with the phenotypical consequences and tissue specificity.
4.4 MeCP2 and the Rett Syndrome
Described in 1966, the Rett syndrome (OMIM # 312750) is a rare disease (prevalence 4/100,000), dominant and X-linked predominantly affecting women. It is characterized by a normal psychomotor development until the age of 6–18 months and the emergence of learning disabilities, autistic behavior, stereotyped hand movements and encephalopathy due to a progressive neurological dysfunction [174]. Demonstrated for the first time in 1999, the causal Rett syndrome mutations are located in the MECP2 gene on Xq28 [175]. MECP2 is ubiquitously expressed, but its highest expression level was found in the brain and fluctuates with neuronal maturation [176, 177]. Most of the pathogenic mutations affect the Methyl Binding (MBD) and Transcription Repression Domains (TRD), and numerous reports have clearly demonstrated that loss or altered expression of the MeCP2 methyl-binding protein leads to the neurological defects observed in the Rett syndrome. Nevertheless, the mechanisms underlying the Rett phenotype are not completely understood. MeCP2 protein might be involved in the epigenetic regulation of a number of genes during neuronal development and maturation such as BDNF but also imprinted genes such as DLX5 and 6 (Distal-Less Homolog) or UBE3A [178–180]. MeCP2 is involved in the global organization of chromatin and long distance looping, in the repression of endogenous retrovirus transcription [178, 181] but also as a modulator of alternative splicing with the RNA binding protein YB1 (Y-Box Binding Protein 1) [182]. In addition, MECP2 binds non-methylated DNA and may act as a transcriptional activator through its interaction with CRB1 at the promoter of target genes [182]. More recently, In vitro and in vivo approaches demonstrated that MeCP2 requires methylated CpGs flanked by a run of at least four A/T for efficient DNA binding conferring to MeCP2 its target specificity and explaining why only a few genes are dysregulated in Mecp2-deficient mice.
Cases with duplication of MECP2 are characterized by severe motor dysfunction, mental retardation, and premature death, indicating that MECP2 dosage is finely regulated in neuronal tissues.
4.5 The Brachydactyly and Mental Retardation Syndrome
The brachydactyly and mental retardation syndrome is linked to mutations in the gene encoding the HDAC4 type IIA histone deacetylase (OMIM # 600430). This pathology linked to deletion of the 2q37 locus, is a rare disorder characterized by a wide clinical spectrum involving developmental delay, skeletal abnormalities, such as brachydactyly type E, facial dysmorphia, and inconsistently, heart defect. This syndrome affecting 1/10,000 individuals (Orphanet) is also characterized by mental retardation and autism. The HDAC4 protein deleted in this pathology acts as a corepressor of transcription and is composed of a N-terminal domain that interacts with transcription factors and a C-terminal nuclear export signal allowing the phosphorylation-dependant transfer of the protein from the cytoplasm to the nucleus [183]. In particular, HDAC4 is a corepressor of transcription factors such MEF2C and RUNX2 and plays a key role in the regulation of genes involved in the osteogenic, chondrogenic, myogenic, and neurogenic differentiation pathways [184–187]. In the central nervous system, HDAC4 protects neurons from apoptosis [113] but might also regulate a number of key genes implicated in the neuronal differentiation pathway suggesting that mutation in the HDAC4 gene modifies expression of a number of genes involved in brain function and neuronal survival.
4.6 The Kleefstra Syndrome or 9q Subtelomeric Microdeletion Syndrome
Kleefstra syndrome (KS; MIM 610253) characterized by severe intellectual disability, hypotonia, microcephaly, epilepsy, and development defects [188, 189], is caused by microdeletions in the distal long arm of chromosome 9q and haploinsufficiency or intragenic loss of function mutations in the gene encoding the EuHMTASE 1/GLP involved in the deposition of Lysine 9 residues on histone H3 to euchromatin and gene repression of individual genes [190–192]. This was established by identification of three patients with features of the syndrome and carrying either mutations or balanced translocation in EHMT1 in which deletion size does not correlate with disease severity since patients with mutations in EHMT1 are as severely affected as those with 9qter submicroscopic deletions encompassing other genes. The role of EHMT1 in the brain and in the etiology of KS is not fully understood but recent data obtained in Drosophila Melanogaster suggest a role for this protein in learning and memory [193]. Ehmt1 +/− mice also exhibit learning and memory deficits associated with a significant reduction in dendritic arborization and number of mature spines in hippocampal CA1 pyramidal neurons [194]. The genes targeted by this histone methyltransferase are not known but EHMT1 haploinsufficiency might be involved in the learning deficits and synaptic dysfunction observed in patients with Kleestra syndrome.
4.7 The Weaver Syndrome: Mutations in EZH2 or NSD1
Type 2 Weaver syndrome (OMIM # 614421) is a rare condition characterized by an abnormal over-growth, tall stature, advanced bone age, a marked macrocephaly, hypertelorism, facial dysmorphism, mental retardation, and learning disabilities [195]. This pathology, mostly sporadic, is caused in some patients by heterozygous mutations in the EZH2 gene on chromosome 7q36.1 [196]. EZH2, Enhancer of Zeste 2 encodes a member of the Polycomb Repressive Complex 2 (PRC2), which catalyzes the trimethylation of lysine 27 of histone H3 (H3K27). As described above, the EZH2 enzyme together with the other members of the PRC2 complex, SUZ12 and EED are involved in the control of cell differentiation, cell lineage determination, stem cell maintenance, X-chromosome inactivation, or genomic imprinting. EZH2 mutations found in the Weaver syndrome cause a loss of the catalytic activity. Furthermore, somatic EZH2 mutations are also encountered in different types of cancers including hematological malignancies [197, 198] suggesting that the congenital mutations found in the Weaver syndrome might also contribute to the increased incidence of cancers, in particular acute lymphoblastic leukemia, observed in this syndrome.
Moreover, in some patients affected with the Weaver syndrome, mutations in the NSD1 gene (nuclear receptor SET domain containing protein–1 gene; OMIM # 117550), which encodes a H3K36 and H4K20 histone methyltransferase have also been observed [199–201]. The NSD1 protein contains a Su(Var)3-9, enhancer of zeste, trithorax (SET) domain responsible for its histone methyltransferase activity, a multiple plant homeodomain (PHD) that associates with chromatin and a proline-tryptophan-tryptophan-proline (PWWD) involved in protein–protein interactions. NSD1 methylates H3K36 and H4K20 in vitro but also non-histone proteins conferring to NSD1 a versatile role in both activation and repression of transcription [202], but its specific function in the brain remains partly understood.
4.8 Epigenetics and Autism
Autism spectrum disorders (ASDs) are a range of complex neurodevelopmental disorders with significant overlap with a number of diseases. ASDs are characterized by repetitive and stereotyped behavior, limited social development and impaired language skills and higher cognitive functions. Cortical organization and circuitry requires coordination of a number of developmental processes such as specification of neuronal identity, neuronal migration, and wiring of neuronal circuits regulated by a number of transcription factors and epigenetic changes occurring at different developmental steps. Because ASD is an early onset neurodevelopmental disorder, alterations during neocortex development are likely involved in the pathology. Furthermore, evidence from recent genetic studies suggests that synaptic dysfunction underlies ASD. In most cases, the etiology is poorly understood and likely involves polygenic factors but also environmental influence and epigenetic dysregulations as exemplified by discordance in monozygotic twins [203]. The involvement of epigenetics is reviewed and discussed here.
As discussed earlier, the MeCP2 protein plays a key role in CNS development. A correlation between reduced MeCP2 expression and autism has been described. Decreased MeCP2 levels have been found in the postmortem frontal cortex samples from autistic patients and in the fusiform gyrus involved in face processing. In the different samples analyzed, decreased MeCP2 expression is associated with hypermethylation of its promoter region suggesting that aberrant DNA methylation causes MeCP2 silencing [140]. However, considering the multiple roles of this protein, its targets and its role either as a silencer or activator of transcription is not known. Nevertheless, it is worth-noting that MeCP2 is linked to the Rett syndrome, classified as ASD.
Beside MeCP2, Methylation changes in ASD have been observed in a certain number of other genes. Their respective roles in disease onset or penetrance are not fully understood and the mechanisms leading to this localized hypermethylation are unknown. In ASD postmortem brain tissues, hypermethylation correlated with decreased expression has been described in the promoter region of the Oxytocin receptor involved in social memory and ability to recognize other individuals [204, 205], the Bcl2 gene [206], the RORA gene (retinoic acid-related orphan receptor alpha) [207], β Catenin [208, 209], or Protein kinase C beta gene (PRKCB1) [210]. Moreover, in a recent study based on 19 autism cases and 21 unrelated controls, methylation level of 485,000 CpG island has been determined using the Infinum HumanMethylation450 BeadChip. Four differentially methylated regions (DMR) have been identified [211]. One of these DMR is located in the 3′ UTR of the PRRT1 gene and overlaps with DNAseI hypersensitive sites. Little is known on the role and regulation of PRRT1 in human but it might be involved in hippocampus function and mutations of other genes of the PRRT family have been observed in a number of neurological disorders. The second DMR was observed in cerebellar tissue, in the promoter of the SDHAP3 gene, associated with a noncoding RNA and a small coding RNA of unknown function. The third DMR identified is located in the promoter region of TSPAN32 and C11orf21. TSPAN32 is involved in cellular immunity and acts as a structural and cell signaling scaffold protein resembling other tetraspanins identified as implicated in schizophrenia and bipolar disorders. Interestingly, this DMR is located within an imprinted region of the genome. The fourth DMR is located upstream of the ZFP57 and overlaps with the 5′ end of an alternatively spliced EST. Interestingly, ZFP57 is instrumental in targeting DNA methyltransferase to imprinted regions during development. As described above, a number of other imprinted loci have been associated with autism suggesting a key role for parent-of-origin methylation in ASD.
Another regulatory pathway possibly affected by epigenetic changes in ASD is the Neurexin-Neuroglin pathway. A large number of mutations have been described in genes such as neuroligins, neurexins, and SHANK that play a role in the formation and the maintenance of synapses. SHANKs are scaffolding proteins of the postsynaptic density of glutamatergic synapses. Mutations or CNVs in the SHANK3 gene have been associated with the Phelan–McDermid syndrome microdeletion syndrome (PMS) in which autistic features are prominent, but also in ASD [212, 213] and schizophrenia associated to intellectual disability (ID) and poor language [214]. The 5′ promoter region of the SHANK3 gene contains five CpG islands and one specific CpG island (number 2) modulates SHANK3 expression in a tissue-dependent manner [215]. The isoform-specific expression of SHANK3 is altered in ASD brain tissues with SHANK3 hypermethylation and treatment with the 5-aza-cytidine DNMT inhibitor modifies the level of SHANK3 methylation together with the isoform-specific expression in cultured cells [216]. Interestingly, the SHANK3 gene is well conserved from rodents to human and the neonatal expression of certain transcripts decrease the methylation of CpG island 2 [217] suggesting a methylation-dependent SHANK3 regulation during development. The cause of increased methylation in specific SHANK3 CpG islands in ASD brain tissues is not known but these observations support an association between hypermethylation, expression changes, and susceptibility to ASD.
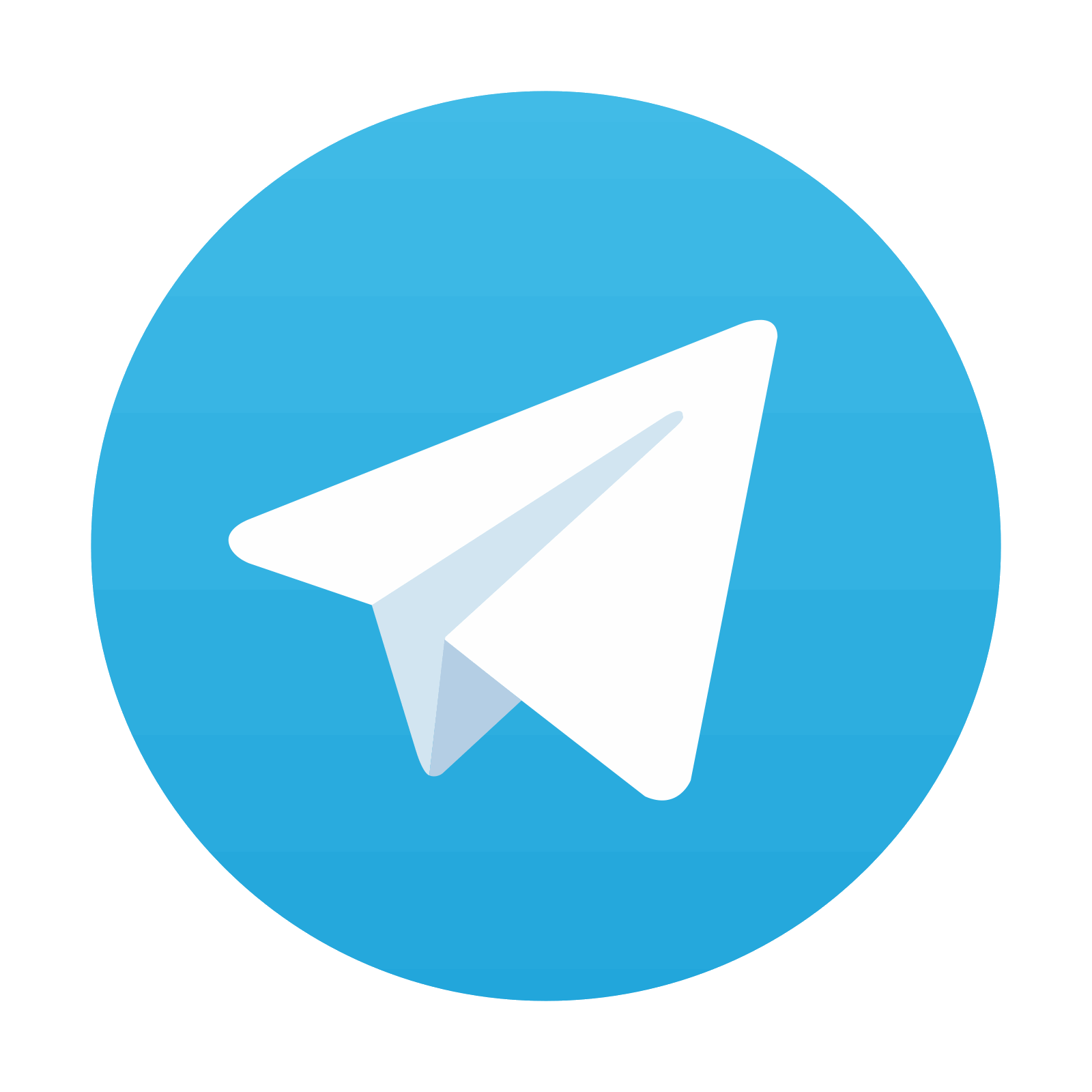
Stay updated, free articles. Join our Telegram channel
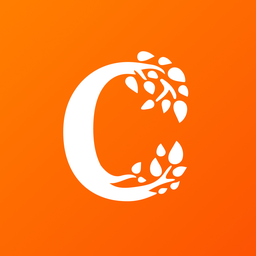
Full access? Get Clinical Tree
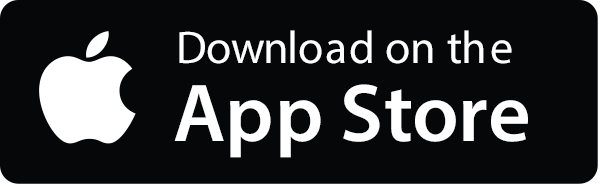
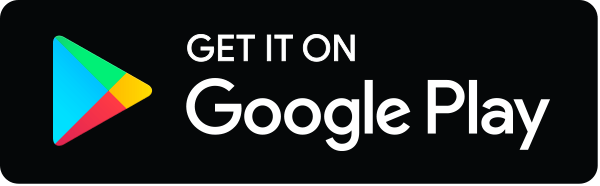