Fig. 5.1
The Epigenome, from nucleus to nucleosome. Schematic illustration of gene (green) poised for transcription by polymerase II (Pol II) initiation complex, with nucleosome free interval at transcription start site (TSS). distal enhancer sequence (blue) which in loop-like structure moves in close proximity to active gene. Subset of heterochromatic portions of the genome (red), including silenced genes, border the nuclear envelope and pore complex and the nucleolar periphery. A small subset of representative histone variants and histone H3 site-specific lysine (K) residues at N-terminal tail (K4, K9, K27, K36, K79) and H4K20 residue are shown as indicated, together with panel of mono- and trimethyl, or acetyl modifications that differentiate between active promoters, transcribed gene bodies, and repressive chromatin, as indicated. DNA cytosines that are hydroxymethylated at the C5 position are in the nervous system most prominent at active promoters and gene bodies, while methylated cytosines are positioned around repressed promoters and in constitutive heterochromatin, and within the body of some of the actively transcribed genes. Specific examples of chromatin regulatory proteins associated with monogenic forms of neurodevelopmental disorders, including autism are provided. For an updated listing of the > 50 genes encoding chromatin regulators and neurodevelopmental risk genes, which includes not only (i) various regulators of DNA methylation and histone PTM and variants, but also (ii) multiple components of the cohesin complex which tethers together promoter-enhancer and other types of chromosomal loopings, and (iii) multiple members of the BAF nucleosome sliding/chromatin remodeling complex, see (Ronan et al. 2013)
DNA (Hydroxy)-Methylation:
Two related but functionally very different types of DNA modifications, methylation (m) and hydroxymethylation (hm) of cytosines in CpG dinucleotides, provide the bulk of the epigenetic modifications in vertebrate DNA (Kriaucionis and Heintz 2009). There are additional types of DNA modifications, which are mostly chemical intermediates in the context of mC5 and hmC5 (cytosines methylated at the carbon 5 position) synthesis and breakdown (Ito et al. 2011). While the majority of DNA (hydroxy)-methylation is found at sites of CpG dinucleotides and, more generally, in the CpG enriched sequences of the genome, a recent study in rat cerebral cortex reported that up to 25 % of mC5 in brain is found at nonCpG sites, a fraction that is far higher than previously assumed (Xie et al. 2012). The mC5 and hmC5 markings show a differential (but not mutually exclusive) pattern of genomic occupancy. The hmC5 mark is concentrated towards the 5’ end of genes and the proximal most portion of transcriptional units, and broadly correlates with local gene expression levels (Jin et al. 2011; Song et al. 2011). There is increasing evidence that the genome-wide distribution of hmC5 is regulated in tissue-specific manner. For example, in brain, one of the tissues with highest levels of hmC5, the mark is enriched in many active genes (Mellen et al. 2012) and could play a role in the regulation of intron/exon boundaries and splicing events of neuron-specific gene transcripts (Khare et al. 2012). Some of these findings in brain resonate with observations on the hmC5 distribution in embryonic stem cells. For example, (Wu et al. 2011) performed genome-wide profile of 5hmC in both wild-type and Tet1-depleted mouse embryonic stem (ES) cells, and their data suggest that 5hmC is enriched at both gene bodies of actively transcribed genes and extended promoter regions of Polycomb-repressed developmental regulators. Furthermore, there is evidence that hmC5 is also enriched in enhancer region marked by H3K4me1 and H3K27ac in human embryonic stem cells (Stroud et al. 2011; Szulwach et al. 2011).
On the other hand, less than < 3 % of methyl-cytosine (mC5) markings are positioned around the 5’ end of genes (Maunakea et al. 2010). The classical concept on the transcriptional regulatory role of DNA methylation , which also has guided many brain related studies, is that promoter-bound repressive chromatin remodeling complexes negatively regulate transcription (Sharma et al. 2005). There are many studies that report changes in promoter DNA methylation (mostly in conjunction with decreased gene expression) in preclinical models of psychosis, depression and addiction, as well as in brain tissue in subjects diagnosed with one of these conditions. Interestingly, however, while the largest amount, or 97 %, of mC5s are found in intra- and intergenic sequences and within DNA repeats (Maunakea et al. 2010), only few of these studies have explored brain DNA methylation changes at repeat DNA and other sequences outside of promoters.
Histone Modifications:
The epigenetic regulation of chromatin by virtue of chemical histone modifications is even more complex than DNA methylation discussed above, and it is now thought that there are far more than 100 amino acid residue-specific post-translational modifications (PTMs) in a typical vertebrate cell (Tan et al. 2011), including mono (me1), di (me2)- and tri (me3) methylation, acetylation and crotonylation, polyADP-ribosylation and small protein (ubiquitin, SUMO) modification of specific lysine residues, as well as arginine (R) methylation and ‘citrullination’, serine (S) phosphorylation, tyrosine (T) hydroxylation, and several others (Kouzarides 2007; Taverna et al. 2007; Tan et al. 2011) . These site- and residue-specific PTMs are typically explored in the context of chromatin structure and function, with an epigenetic histone code (a combinatorial set of histone PTMs that differentiates between promoters, gene bodies, enhancer and other regulatory sequences, condensed heterochromatin, and so on) (Zhou et al. 2011). It is important to emphasize that histone PTMs rarely occur in isolation, and instead multiple histone PTMs appear to be co-regulated and, as a group, define the aforementioned chromatin states (Berger 2007). Many active promoters, for example, are defined by high levels of histone H3 lysine 4 methylation in combination with various histone lysine acetylation markings (Zhou et al. 2011). Repressive histone PTMs, including the trimethylated forms of H3K9, H3K27 and H4K20, potentially co-localize to some of the same loci in the genome, and so forth. Furthermore, there is also evidence for a coordinated and sequential regulation; phosphorylation of histone H3 at the serine (S)10 position often serves as a trigger for subsequent acetylation of neighboring lysine residues histone H3 lysine 9 (H3K9) and lysine 14 (H3K14) in the context of transcriptional activation, while at the same time blocking repression-associated methylation of H3K9 (Nowak and Corces 2004). Of note, proteins associated with the regulation of histone PTMs are sometimes referred to as ‘writers’, or ‘erasers’ or ‘readers’, essentially differentiating between the process of establishing or removing a mark as opposed to its docking functions for chromatin remodeling complexes that regulate transcription, or induce and maintain chromatin condensation (Taverna et al. 2007; Mosammaparast and Shi 2010; Justin et al. 2010). Obviously, the concept of chromatin ‘reader’, ‘writer’ and ‘eraser’ proteins could easily be expanded to proteins associated with DNA methylation (Fig. 5.1) .
Histone Variants:
In addition to the core histones H2A/H2B/H3/H4, histone variants such as H3.3, H2A.Z and H2A.X exist . The role of these variant histones, which differ from the canonical histones only at very few amino acid positions, is often discussed in the context of replication-independent expression and assembly (Woodcock 2006), and several histone variants robustly affect nucleosome stability and compaction (Jin and Felsenfeld 2007). One popular model postulates that during the process of gene expression, RNA polymerase and the transcriptional activation and elongator complexes destabilize nucleosomes, which in turn promotes nucleosome remodeling and variant histone incorporation which then further potentiate or stabilize gene expression (Sutcliffe et al. 2009; Bintu et al. 2011) .
Chromatin-Bound RNAs (CBRs):
While the process of gene expression is obviously defined by nascent RNA emerging from genomic DNA packaged into chromatin, the term CBR could be reserved to RNA species as part of a chromatin structure, thereby regulating its functions. None of these definitions are mutually exclusive, however. According to some estimates, up to 2–3 % of the nucleic acid content in chromatin is contributed by polyadenylated RNAs (Rodriguez-Campos and Azorin 2007). One of the best known examples of a CBR is provided by the X-chromosome Inactive Transcript (XIST) (Brockdorff 2013; Zhao et al. 2008).
Perhaps one of the most illustrative and complex examples as it pertains to ASD-associated CBR involves chromosome 15q11-13, a highly regulated locus, subject to genomic imprinting (parent-of-origin-specific gene expression) and responsible for a range of neurodevelopmental syndromes, including Prader-Willi and Angelman, as well as for a subset of cases diagnosed with ASD (Leung et al. 2011). Furthermore, DNA structural variants within this locus could contribute to genetic risk to schizophrenia and bipolar disorder, further emphasizing that this locus is broadly relevant for a range of neuropsychiatric disease (Leung et al. 2011). Of note, a very large ncRNA arises from 15q11-13, covering 1 Mb in the mouse and 600 kb in humans and 148 exons and introns (Le Meur et al. 2005). This long SNPRN-UBE3A ncRNA, which normally is highly expressed on the paternal but not on the maternal chromosome, includes clusters of smaller non-coding RNAs that are thought to modulate nucleolar functions in neurons, and an antisense transcript, UBE3A-AS which suppresses UBE3A sense transcription of the same gene on the paternal chromosome (Leung et al. 2011). Evidence has been that the SNPRN-UBE3A ncRNA, and the smaller RNAs derived from it, produce a ‘RNA cloud’ in cis, which contributes to lasting decondensation of this locus on the paternal chromosome, including epigenetic decoration with open chromatin-bound histone modifications and loss of repressive chromatin-associated histone and DNA methylation (Leung et al. 2011; Xin et al. 2001). Interestingly, UBE3A (also known as E6-AP) encodes a ubiquitin ligase that targets RING-1B, a component of Polycomb repressive complex PRC1, for its subsequent degradation (Zaaroor-Regev et al. 2010). Because PRC1 is a key regulator for genome-wide repressive histone (H3K27) methylation, dysregulated expression of long SNPRN-UBE3A ncRNA in the context of 15q11-13 imprinting disorders and/or genetic mutations and polymorphisms, may affect orderly activity of the PRC1 complex in developing brain (Vogel et al. 2006; Tarabykin et al. 2000; Golden and Dasen 2012), perhaps resulting in chromatin defects across widespread portions of neuronal or glial genomes, with serious implications for brain function and behavior.
Chromatin Remodeling and Nucleosome Positioning:
Chromatin remodeling complexes are comprised of multiple subunits, that according to their classical definition regulate sliding and mobility of nucleosomes , powered by ATP hydrolysis, thereby regulating gene expression and RNA polymerase II access at transcription start sites (Ronan et al. 2013). Examples of well known chromatin remodelers with a critical role in brain development include the BAF (SWI/SNF) complex and CHD family of proteins (Ronan et al. 2013). Interestingly, mutations in numerous members of the BAF complex and multiple CHD proteins have now been linked to psychiatric disease and developmental brain disorders (Ronan et al. 2013).
Higher Order Chromatin Structures:
Epigenetic decoration of nucleosomes, including the DNA and histone modifications, and histone variants described above, in itself, would fall short to adequately describe the epigenome , or even the localized chromatin architecture at any given (genomic) locus. This is because nucleosomal organization leads to only a 7-fold increase in packaging density of the genetic material, as compared to naked DNA; however, the actual level of compaction in the vertebrate nucleus in interphase (which defines the nucleus during the time period a cell is not dividing, including postmitotic cells such as neurons) is about three orders of magnitude higher (Belmont 2006). The chromosomal arrangements in the interphase nucleus are not random, however. Specifically, loci at sites of active gene expression are more likely to be clustered together and positioned towards a central position within the nucleus, while heterochromatin and silenced loci move more towards the nuclear periphery (Cremer and Cremer 2001; Duan et al. 2010). Chromosomal loopings, in particular, are among the most highly regulated ‘supranucleosomal’ structures and are associated with transcriptional regulation, by, for example, positioning distal regulatory enhancer or silencer elements that—in the linear genome—are positioned potentially many hundred of kilobases apart from a gene, to interact directly with that specific promoter (Wood et al. 2010; Gaszner and Felsenfeld 2006).
The regulation of higher order chromatin is certainly of critical importance for human health, including orderly brain development and function. For example, Cornelia de Lange Syndrome (CdLS) with an estimated incidence of 1:10–30,000 live births among the more frequent genetic disorders (source http://ghr.nlm.nih.gov) is associated with severe developmental delay and a range of neuropsychiatric symptoms, including ASD and psychosis (Moss et al. 2008). CdLS (including Online Mendelian Inheritance of Man (OMIM) 122470 and 300590) involves causative mutations in the cohesin complex, a multisubunit protein that includes, among others, nipped B-like protein (NIPBL), structural maintenance of chromosomal proteins SMC1A and SMC3, and histone deacetylase HDAC8 (Deardorff et al. 2012; Gervasini et al. 2013). Cohesin is thought to form ring-like structures bringing together DNA segments from different locations, and by interaction with transcriptional co-activators such as the Mediator multi-protein complex, these protein networks could provide the foundation for chromosomal loop formations, including promoter-enhancer loopings that define cell-type specific gene expression programs (Kagey et al. 2010).
Thus, there can be little doubt that epigenetic regulation is of critical importance for orderly brain development. The list of chromatin regulators (Ronan et al. 2013) includes not only methyl-CpG-binding proteins (incl. MECP2, the Rett Syndrome gene) but also multiple members of the BAF and also CHD complexes regulating nucleosome mobility (Ronan et al. 2013). Another pathway with multiple genes found to have mutations in larger ASD cohorts includes H3K4 methyltransferases and demethylases MLL1 (Jones et al. 2012), MLL2 (Hannibal et al. 2011), MLL3 (O’Roak et al. 2012; Kleefstra et al. 2012; Neale et al. 2012), and KDM5A, KDM5C/JARID1C/SMCX (Adegbola et al. 2008; Najmabadi et al. 2011; Jensen et al. 2005). As we pointed out above, these detailed findings from clinical genetics will require further workup of the cell type(s) and developmental stage(s) at risk, because the neurobiology of disease driven by these genes and their mutations remains essentially unresolved.
5.2 ‘Epigenetic Heritability’ in ASD?
Significant strides have been achieved in unraveling the genetic risk architecture of ASD . Thus, it is now estimated that up to 30 % of cases harbor either (i) identifiable chromosomal microdeletions and duplications (copy number variants) that often encompass many hundreds of kilobases of sequence on the linear genome (7–20 % of cases), or (ii) single gene disorders such as Fragile X, Rett Syndrome and others that in toto account for 5–7 % of all cases, and another (iii) 5 % of cases are due to metabolic diseases including mitochrondrial disease, phenylketonuria, adenylosuccinate lyase deficiency etc (Schaaf and Zoghbi 2011). This still leaves 70 % of cases with no straightforward genetic explanation, albeit genetic approaches such as whole exome and whole genome sequencing are beginning to make additional inroads in the complex field of ASD genetics, mostly by identifying additional rare variants of potentially high disease risk (Gratten et al. 2013; Shi et al. 2013) . There are also ongoing refinements in mapping some of the common (mostly single nucleotide) polymorphisms that contribute to the genetic risk of ASD and other psychiatric and neurodevelopmental disorders (Smoller et al. 2013).
In addition to the above described genetic risk structure of ASD—there is both indirect and direct evidence for epigenetic component(s) in the etiology of the disorder. At the population level, twin studies have almost exclusively been used to demonstrate substantial genetic contribution to ASD etiology. However, it is important to underscore that the concordance rates are not exceeding 50 % in monozygotic twin studies in ASD and related disease (Steffenburg et al. 1989; Bailey et al. 1995; Chang et al. 2013)—providing ample evidence for the role of environmental factors, and for epigenetic processes in the etiology of ASD. The framework for the epigenetics of ASD includes an increasing list of deleterious mutations and DNA structural variants directly resulting in dysregulated chromatin, including several portions of chromosomes 15q and 7q that are classical sites for genomic imprinting, as defined by allele-specific DNA methylation signatures differentially regulated on the maternally vs. paternally derived chromosomes (Schanen 2006). Therefore, it is entirely possible that disruption of gene expression via epigenetic mechanisms not reflected in the primary nucleotide sequence, or so called ‘epialleles’, either due to de novo dysregulation in very early development, or by inheritance and carry-over of epigenetic information through the parental germline, plays an important role in some causes of ASD and other psychiatric disorders .
Here, we define epigenetic heritability simply as a phenotype or predisposition that is transmitted from one generation to the next, by some sort of molecular information in the germ cell, that is not encoded by the base pair DNA sequence of the (transmitted) genome. It is often speculated that ‘abnormal’ epigenetic decoration of the haploid germ cell genome (including DNA and histone modifications, see below) contribute to heritable risk of common (including psychiatric) disorders but convincing evidence, or even proof, of ‘true epigenetic heritability’ in disorders such as ASD is extremely difficult to accomplish with present day technologies, because it would, among others, require demonstration that DNA structural variants either in cis (at the site of the epigenetic defect) or trans (other locations in the genome) were not driving the chromatin alterations. Nonetheless, there is an increasing amount of correlative evidence in support of epigenetic heritability in humans, including the role of the ancestral nutritional environment in metabolic disease risk in children and grandchildren (Rando 2012). In the following sections, we summarize some of the most interesting findings as it pertains to the possibility for disease-relevant epigenetic heritability :
1.
Nucleosomal organization and DNA:histone octamer packaging is retained in 4 % of the haploid genome in sperm, and these retained nucleosomes are enriched significantly at loci of developmental importance, including imprinted gene clusters, microRNA clusters, homeobox (HOX) gene clusters, and the promoters of stand-alone developmental transcription and signaling factors (Hammoud et al. 2009). Furthermore, DNA methylation patterns and histone modifications are also preserved in the portion of the genome that maintains nucleosomal organization in sperm cells (Hammoud et al. 2009; Okada et al. 2010). The limitations of present day genome technology, including the lack of genome-wide high quality sequence information when only few cells are available as input has so far prevented our ability to map the epigenome of human oocytes (McGraw et al. 2013); however, high quality DNA methylation maps have also been established for mouse oocytes (Shirane et al. 2013; Smallwood et al. 2011). Therefore, in principle, chromatin templates, including their epigenetic markings, could be passed on to the next generation for perhaps as much as 4 % of the human genome (Hammoud et al. 2009), and therefore the portion of the genome subject to transgenerational epigenetic inheritance would indeed be larger than the entire protein coding sequence (‘exome’) which amounts to ‘only’ 1–1.5 % of the genome.
2.
Paternal age, (and specifically advanced parental age), has been linked to an increased risk for neurodevelopmental disorders, including ASD, and much of this effect could be attributed to an age-associated risk of rare de novo mutation, with a doubling of the mutational burden through the paternal age every 16 years, according to some of the more widely publicized findings (Kong et al. 2012). Twin studies have demonstrated that advancing paternal age modifies twin concordance for autism (Lundstrom et al. 2012). There is evidence that advanced grandpaternal age contributes independently to increase the risk for ASD by approximately 1.7-fold both for the maternal and paternal lines (Frans et al. 2013) . Preclinical work in mice and rat would suggest that, at least for the paternal age effect, epigenetic mechanism could play a role too. Thus, brain of offspring from older fathers showed DNA methylation abnormalities at multiple imprinted gene loci (imprinted loci show parent-of-origin specific epigenetic regulation and are by some authors considered to be more sensitive to epigenetic pertubations) (Smith et al. 2013). In addition, aberrant DNA methylation in sperm of older animals has been observed at DNA repeats encoding ribosomal genes (Oakes et al. 2003).
3.
In the rodent, there is ample evidence for transgenerational effects of environmental influences, including nutrition, stress and drugs, impacting brain and behavior for at least one, or few generations. These involve DNA methylation and histone modification changes in a number of molecules involved in serotonergic signaling and neurotrophins (including brain-derived neurotrophic factor (Bdnf)) (Bohacek and Mansuy 2013; Morgan and Bale 2011; Vassoler et al. 2013).
4.
In humans, adverse environmental conditions, including famine around the time of conception, have been linked to lasting DNA methylation changes at the site of some of the best studied imprinted gene loci, including IGF2R/H19 (Heijmans et al. 2008) and could contribute to the increased risk for neurodevelopmental and also endocrine disorders that are associated with these conditions, including diabetes and schizophrenia (Lumey et al. 2011) .
5.
Maternal prenatal use of prescribed medications has been recently associated with risk for ASD. Risks for ASD have been reported for selective serotonin reuptake inhibitors (SSRI) and other monoamine reuptake inhibitors (Rai et al. 2013), and mood stabilizers such as valproate (Meador and Loring 2013; Christensen et al. 2013). Psychiatric and neurologic medications are used in > 3 % of women of child-bearing potential, all cross the placenta and animal models of their exposure result in aberrant neurodevelopment (Walsh et al. 2008; Pardo and Eberhart 2007). Adverse effects of these drugs include interference in GABAergic, dopaminergic, serotoninergic, and glutamatergic pathways. Importantly, they have been shown to effect DNA methylation (Mill et al. 2008; Tsankova et al. 2007; Melas et al. 2012) and histone modifications (Peter and Akbarian 2011; Li et al. 2004).
5.3 Monogenic Forms of ASD Due to Mutations in Genes Encoding Chromatin Regulatory Proteins
The list of mutations carrying a high risk for ASD, even as singular genetic factors, includes an increasing number of genes encoding a chromatin regulatory protein . This is not too surprising, because chromatin remodeling and proper assignment of epigenetic marks is of fundamental importance for brain ontogenesis and a key control point in the stepwise transition from pluripotency to neural precursor to terminally differentiated neurons and glia (Ho and Crabtree 2010), and involved in developmental events such as neuronal migration and connectivity formation (Fuentes et al. 2011). On the other hand, ASD-associated chromatin disorders were initially known only in the context of embryonic defects and multi-organ syndromes, with some of the more recently discovered gene defects affecting selectively the brain without significant involvement of peripheral organs. Well known examples of the former type of gene defects include Rubinstein-Taybi syndrome (RSTS) 1 (Online Mendelian Inheritance of Man, OMIM 180849) and 2 (OMIM 613684) (Roelfsema and Peters 2007), as well as the Immunodeficiency, centromere instability, facial anomalies (ICF1) mental retardation syndrome (OMIM 242860) (de Greef et al. 2011; Ehrlich et al. 2008), among others. The most well known example of the latter type of gene defects includes Rett Syndrome, an X-linked neurological disorder of early childhood (OMIM 312750). To date, deleterious mutations in over 50 different chromatin regulators have been associated with psychiatric disorders including ASD and schizophrenia (Ronan et al. 2013) .
5.4 Epigenetic Regulation in the Human Brain During Normal Development and Aging—Implications for ASD
Chromatin remodeling and epigenetic mechanisms are involved in developmental events such as neuronal migration and connectivity formation (Fuentes et al. 2011) . Furthermore, there is increasing evidence that the normal course of maturation and aging is associated with changes in the brain’s epigenome . On the one hand, this is an attractive hypothesis given that there are widespread age-related changes in gene expression in the cerebral cortex , including downregulation of many neuronal genes (Erraji-Benchekroun et al. 2005; Tang et al. 2009). However, in contrast to the accumulation of somatic mutations and other structural brain DNA changes that affect promoter function during aging (which are likely to be irreversible) (Lu et al. 2004), most or perhaps all epigenetic markings studied to date are now thought to be reversible, and there is no a priori reason for unidirectional accumulation of a specific epigenetic mark in aging brain chromatin. Nonetheless, an increasing body of literature indicates that a substantial reorganization of the epigenome occurs during postnatal development and aging. Human cerebral cortex, for example, shows complex and gene-specific changes in levels of methyl-cytosine (mC5; cytosines are methylated at the carbon 5 position), with a steady rise at many promoters that continues into old age in conjunction with subtle changes (mostly a decline) in gene expression (Siegmund et al. 2007; Hernandez et al. 2011).
The increasing list of monogenic forms of neurodevelopmental disease due to mutations in genes encoding specific chromatin remodelers speaks to the importance of these mechanisms for brain ontogenesis and early maturation. It is interesting to note that DNA and histone methylation signatures show, on a genome-wide scale, the most dramatic changes during prenatal development, and the transition phases from perinatal period to early infancy and the perinatal period; in striking contrast, epigenetic changes during subsequent periods of maturation and aging, including puberty and various phases of adulthood, are comparatively minor (Siegmund et al. 2007; Numata et al. 2012; Shulha et al. 2013; Cheung et al. 2010). Cell-type specific epigenome profilings confirmed that these developmental changes are not explained by the various shifts in neuron-to glia ratios and other changes in cell type composition and instead, point to large scale chromatin remodeling in cortical neurons during prenatal and early postnatal development (Cheung et al. 2010; Shulha et al. 2013; Siegmund et al. 2007).
Thus, one could conclude that immature neurons are, both in utero and for the first few months or years after birth, particularly vulnerable to epigenetic pertubations, and some of these could have played a role in the etiology of some cases on the autism spectrum. This hypothesis is further supported by the observation that prenatal exposure to certain types of drugs, including the short chain fatty acid derivative and histone deacetylase inhibitor, sodium valproate, is associated with a 2–3 fold increase in the risk of the offspring to develop ASD (Christensen et al. 2013). Because epigenetic mechanisms literally serve as a molecular bridge linking the genome to the environment, it is worth noting in this context that a recent large twin cohort attributed the estimated risk of shared in utero environment at 30–80 % for ASD, exceeding the estimated genetic risk of 14–67 % (Hallmayer et al. 2011).
5.5 Gene Expression and Chromatin Alterations in ASD Postmortem Brain
ASD, like many other psychiatric disorders, lacks a unifying neuropathology and is probably comprised of a diverse set of syndromes of considerable genetic and etiologic heterogeneity . Interestingly, however, studies even in fairly small postmortem brain cohorts with typically less than 20 cases and equal number of controls have shown significant, disease-associated changes in RNA and protein levels. For example, there is literature reporting downregulated GABAA and GABAB receptor expression and ligand binding across multiple areas of the cerebral cortex and cerebellum , in conjunction with altered expression of GABA synthesis enzymes and GABA neuron-specific peptides in cerebral cortex, hippocampus and cerebellum, reviewed in (Blatt and Fatemi 2011). As in other conditions, including schizophrenia (Akbarian and Huang 2006), changes in the GABAergic transcriptome appear to affect a significant portion of cases on the autism spectrum, albeit the reported RNA alterations are not sufficiently consistent to reach the level of significance in all of the postmortem studies (Huang et al. 2010). Nonetheless, this work in the clinical tissue samples provided one of the cornerstones for the popular hypothesis that alterations in the balance of excitatory and inhibitory (E/I) activity play a critical role in the pathophysiology of a substantial number of cases on the autism spectrum, affecting cortical inhibitory (GABAergic) circuitry and synchronization of electrical activity across widespread brain regions in the autistic brain (Uhlhaas and Singer 2012; Rubenstein 2010) .
The complete set of gene expression changes in the autistic brain are likely to go far beyond these GABAergic RNAs. For example, recent microarray studies identified a diverse set of neuronal genes and noncoding RNAs, many of which positioned in genomic loci conferring genetic ASD susceptibility and that showed altered expression in prefrontal and temporal cortex or cerebellar cortices (Voineagu et al. 2011; Ziats and Rennert 2013), with additional gene expression changes indicative of a dysfunction of immune regulation in at least some of the disease cases (Voineagu et al. 2011). Given that transcriptional regulation is closely associated with dynamic changes in chromatin structure and function (Lee and Young 2013), it would not be too surprising that these gene expression alterations in the diseased brain are associated with epigenetic changes in cis-regulatory sequences such as transcription start sites.
However, one challenge for epigenetic studies in brain is the enormous cellular heterogeneity of the postmortem tissue, with its mixed population of glutamatergic and GABAergic neurons, mature oligodendrocytes and astrocytes and their precursors as well as endothelial cells. Furthermore, some brains from subjects on the autism spectrum show evidence for inflammation , including activation and proliferation of microglia as the brain’s immune surveillance cells (Theoharides et al. 2013; Suzuki et al. 2013; Tetreault et al. 2012). This is an important problem given that cell-type specific differences in DNA and histone methylation landscapes are, on a genome-wide scale, far greater than, for example, developmentally regulated changes within a specific cell type, or species-specific regulation in the primate brain (Shulha et al. 2013, 2012b; Cheung et al. 2010) . Thus, conventional chromatin assays, which are designed to detect and quantify DNA methylation and histone modifications requiring an input material between 103–108 nuclei (Huang et al. 2006; Adli and Bernstein 2011), could be severly confounded by changes in glia-to-neuron ratios that could show considerable fluctuations across different developmental or disease states. To date, many studies exploring epigenetic dysregulation of gene expression in major psychiatric disorders examined DNA methylation and histone modifications in tissue homogenates, thus ignoring the fact that the gene(s)-of-interest often are expressed only in a select subpopulation of neurons or other cells.
To bypass these limitations, a recent postmortem brain study, conducted on 16 subjects on the autism spectrum and 16 controls of child and adult age, profiled the transcriptional mark, histone H3-trimethylated at lysine 4, on a genome-wide scale in prefrontal cortical neurons and separately, in non-neuronal chromatin from the same cases (Shulha et al. 2012a). The study identified 711 “epigenetic risk” loci were affected in variable subsets of autistic individuals, including the synaptic vesicle gene RIMS3, the retinoic acid signaling regulated gene RAI1 (Nakamine et al. 2008), the histone demethylase JMJD1C (Castermans et al. 2007), the astrotactin ASTN2 (Glessner et al. 2009), the adhesion molecules NRCAM (Sakurai et al. 2006; Bonora et al. 2005; Petek et al. 2001) and SEMA5 (Weiss et al. 2009; Melin et al. 2006), the ubiquitin ligase PARKIN2 (PARK2) (Scheuerle and Wilson 2011; Glessner et al. 2009) and many other genes for which rare structural DNA variations could carry high disease penetrance . Intriguingly, for most of these epigenetic changes, the H3K4me3 changes occurred selectively in prefrontal neurons, but not in their surrounding non-neuronal cells (Shulha et al. 2012a). Therefore, the disease-associated epigenetic signatures in ASD are cell-type specific and, at least in neurons, show significant overlap with the genetic risk architecture of neurodevelopmental disorders (Shulha et al. 2012a).
Another study, using peripheral blood cells from monozygotic twins discordant for ASD, identified multiple disease-associated differentially DNA methylated regions, which would suggest that at least some of the epigenetic alterations in subjects with ASD may be unrelated to the genetic risk architectures in the affected cases (Wong et al. 2013).
5.6 Epigenetic Drug Targets to Treat ASD
To date, no medication has been approved for the treatment of core symptoms of ASD, including social and other cognitive defects and problems associated with language and communication and interaction (Payakachat et al. 2012) . Preliminary data suggest that intranasal application of the nonapeptide, oxytocin , when administered in research and laboratory settings, elicits a modest improvement in repetitive behaviors and some of the test measures for social cognition (Bakermans-Kranenburg and van Ijzendoorn 2013). In addition, antipsychotic medications (originally designed to treat schizophrenia and other types of psychosis) such as risperidone and aripiprazole demonstrated improvement in parent-reported measures of challenging behaviors such as repetitive or aggressive behaviors, but carry a significant side-effect burden (Payakachat et al. 2012). Other types of drugs, including anticonvulsants and anxiolytics may be very beneficial in selected cases to treat seizures and anxiety but obviously do not address the core symptoms. Thus, there is a pressing need to pursue novel and innovative treatment options for these types of conditions. It is not unreasonable to explore promising epigenetic drug targets in ASD .
One recent, impressive example for epigenetic drug targets is provided by topoisomerases (topos), DNA cleaving enzymes that are important in the processes for replication and recombination, transcription and chromatin remodeling (Salerno et al. 2010). A recent study using an unbiased high-content screening approach, using mouse primary cortical neurons, discovered that a diverse group of molecules, that share an inhibitory activity against DNA topoisomerase type I or II enzymes can unlock the expression of the normally epigenetically silenced paternal allele of the gene encoding ubiquitin protein ligase E3A (Ube3a). These topo inhibitors mediate their effect by reducing the expression of the imprinted Ube3a antisense RNA (Ube3a-ATS) (Huang et al. 2011). The expression of this antisense RNA is normally repressed in the maternal chromosome in conjunction with the allele-specific DNA methylation of an imprinting center (a DNA or chromatin structure that carries epigenetic information about parental origin) (Bressler et al. 2001). Similar to hundreds of other loci defined by parent-of-origin-specific gene expression, Ube3a was considered epigenetically stable throughout life (Reik 2007). This hypothesis now needs to be revised, however, given that even a single intrathecal infusion of the FDA-approved topoisomerase inhibitor topotecan was sufficient to relieve silencing of the paternal Ube3a (sense) transcript in lumbar spinal neurons for an extended period of at least 3 months (Huang et al. 2011) . The most obvious explanation for topotecan’s mechanism of action—altered DNA methylation of the Ube3a imprinting center—has been ruled out, thus the underlying mechanism(s) remain a mystery. Thus, the reactivation of paternal UBE3A expression via topoisomerase inhibition could provide a starting point to investigate potential therapies for Angelman syndrome, which is caused by loss of function mutations and deletions at the maternal UBE3A locus (Kishino et al. 1997; Matsuura et al. 1997; Sutcliffe et al. 1997), and for which there are currently no effective treatments. Currently, it remains unknown whether topoisomerase-mediated reversal of imprinting-related gene expression is specific to the UBE3A locus. However, this issue could be addressed by, for example, fusing topoisomerase enzymes to customized motifs for sequence-specific binding at UBE3A. For example, zinc finger nucleases or transcription activator-like (TAL) effectors of plant pathogenic bacteria have been fused to the FokI restriction enzyme, allowing the induction of‘custom-made’ DNA strand breaks at specific and even at unique loci in the genome (Porteus and Baltimore 2003; Bogdanove and Voytas 2011). An even more promising toolkit was recently introduced to the field, utilizing a genome defense mechanism in bacteria, CRISPR (Clustered Regularly Interspaced Short Palindromic Repeats)/Cas (CRISPR-associated) (Bhaya et al. 2011). The Type II CRISPR/Cas system requires a single protein, Cas9, to catalyze DNA cleavage (Sapranauskas et al. 2011) and is now widely used to induce targeted mutations in eukaryotic systems, including human and mouse cells (Cong et al. 2013) .
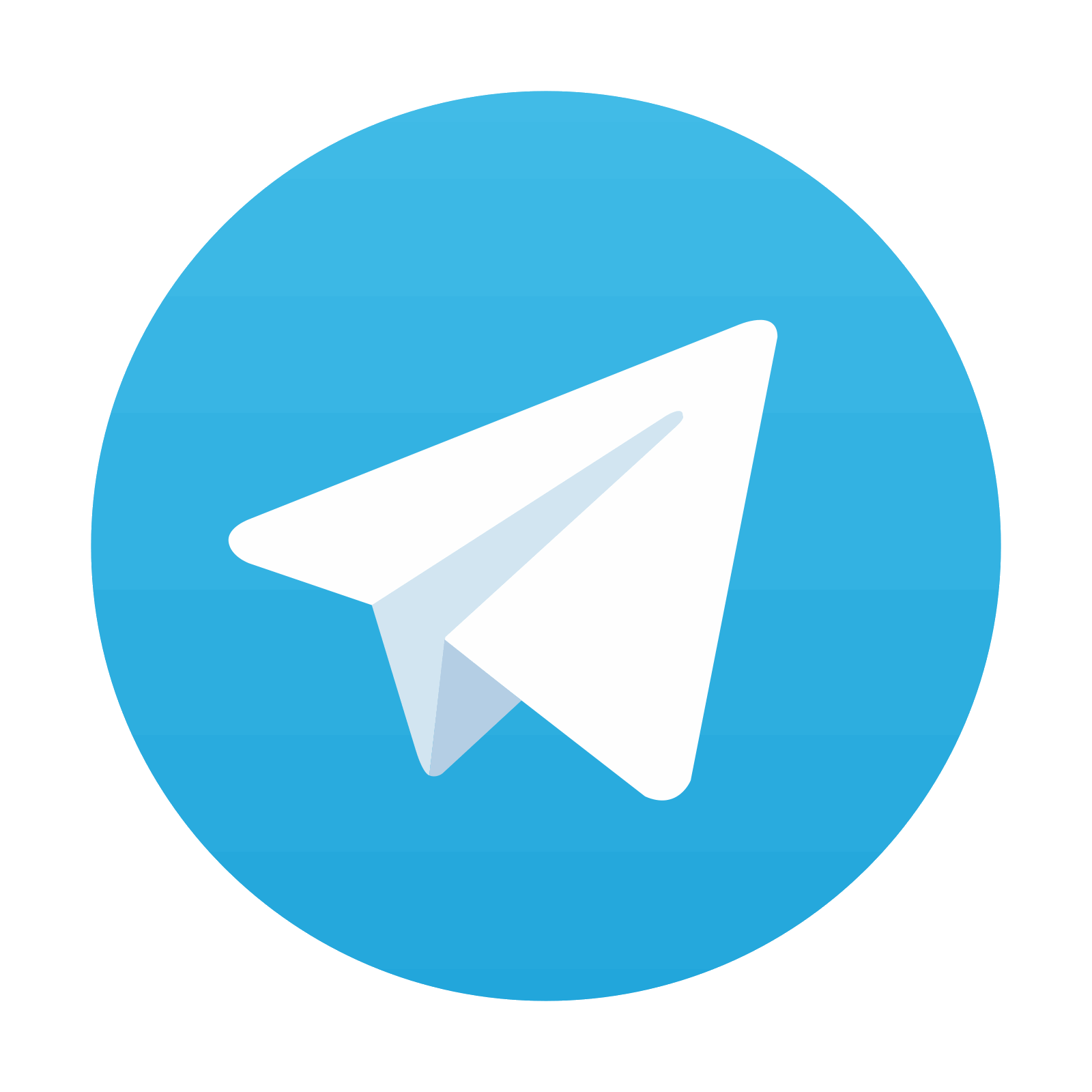
Stay updated, free articles. Join our Telegram channel
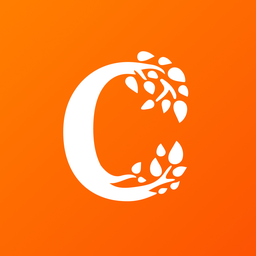
Full access? Get Clinical Tree
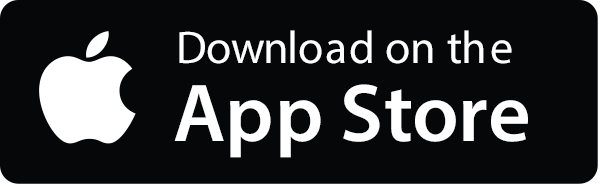
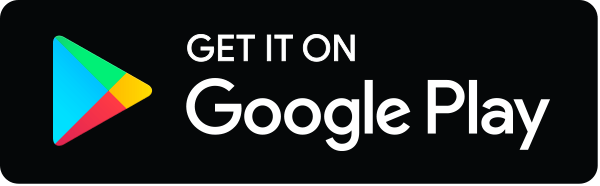