and Wah Chin Boon2
(1)
Mental Health Institute of Victoria, Parkville, VIC, Australia
(2)
Howard Florey Institute, Parkville, VIC, Australia
Abstract
Estrogens are C-18 phenolic steroids derived from cholesterol and occur naturally in the forms of 17β-estradiol, estrone, and estriol. Estrogen biosynthesis begins with the transfer of cystolic cholesterol from the cytoplasm into the mitochondrion of steroidogenic cells (1-3). This transfer of cholesterol into the mitochondrion is facilitated by the steroidogenic acute regulatory protein (StAR) (1). This is the rate-limiting step in androgen biosynthesis, as the cholesterol has to be transferred to the site where the cytochrome P450 side-chain cleavage enzyme (P450scc, CYP11A1) is located. As its name indicates, it catalyzes the cleavage of the side-chain of cholesterol (4) to form pregnenolone. Conversion of pregnenolone to estrogens involves five catalyzing enzymes which, in a number of steps, convert pregnenolone to progesterone, progesterone to androgens, and finally, androgens to estrogens (1). The final and rate-limiting step in estrogen biosynthesis is the aromatization of testosterone and androstenedione to 17β-estradiol and estrone, respectively. Aromatization is catalyzed by the P450 aromatase monooxygenase enzyme complex (P450 aromatase, P450arom, CYP19; (5)). In premenopausal women, estrogens are predominantly formed by aromatization in the ovaries. In comparison to premenopausal women, serum 17β-estradiol concentrations in men and postmenopausal women are low. Most of the 17β-estradiol in the latter is formed by aromatization in extragonadal tissues (6), such as the adipose tissue (7), muscle (7), bone (8), adrenal (9), vasculature, and numerous sites in the brain (10, 11). Other estrogen-synthesizing tissues include testis (12), placenta (13), fetal (but not adult) liver (14). Adipose tissue synthesizes estrone from circulating androstenedione (15) secreted by the adrenal cortex (16). Of these two estrogens, 17β-estradiol is the more biologically active. In the placenta, estriol is synthesized from its precursor, 16α-hydroxydehydroisoandrosterone, and this substrate is derived from the fetal adrenal and liver (15). Estrogens may then exert their actions via estrogen receptor-dependent genomic and nongenomic actions, or possibly by estrogen receptor-independent mechanisms (see review (17)).
1 Estrogen Biosynthesis and Metabolism
1.1 Estrogen Biosynthesis
Estrogens are C-18 phenolic steroids derived from cholesterol and occur naturally in the forms of 17β-estradiol, estrone, and estriol. Estrogen biosynthesis begins with the transfer of cystolic cholesterol from the cytoplasm into the mitochondrion of steroidogenic cells (1–3). This transfer of cholesterol into the mitochondrion is facilitated by the steroidogenic acute regulatory protein (StAR) (1). This is the rate-limiting step in androgen biosynthesis, as the cholesterol has to be transferred to the site where the cytochrome P450 side-chain cleavage enzyme (P450scc, CYP11A1) is located. As its name indicates, it catalyzes the cleavage of the side-chain of cholesterol (4) to form pregnenolone. Conversion of pregnenolone to estrogens involves five catalyzing enzymes which, in a number of steps, convert pregnenolone to progesterone, progesterone to androgens, and finally, androgens to estrogens (1). The final and rate-limiting step in estrogen biosynthesis is the aromatization of testosterone and androstenedione to 17β-estradiol and estrone, respectively. Aromatization is catalyzed by the P450 aromatase monooxygenase enzyme complex (P450 aromatase, P450arom, CYP19; (5)). In premenopausal women, estrogens are predominantly formed by aromatization in the ovaries. In comparison to premenopausal women, serum 17β-estradiol concentrations in men and postmenopausal women are low. Most of the 17β-estradiol in the latter is formed by aromatization in extragonadal tissues (6), such as the adipose tissue (7), muscle (7), bone (8), adrenal (9), vasculature, and numerous sites in the brain (10, 11). Other estrogen-synthesizing tissues include testis (12), placenta (13), fetal (but not adult) liver (14). Adipose tissue synthesizes estrone from circulating androstenedione (15) secreted by the adrenal cortex (16). Of these two estrogens, 17β-estradiol is the more biologically active. In the placenta, estriol is synthesized from its precursor, 16α-hydroxydehydroisoandrosterone, and this substrate is derived from the fetal adrenal and liver (15). Estrogens may then exert their actions via estrogen receptor-dependent genomic and nongenomic actions, or possibly by estrogen receptor-independent mechanisms (see review (17)).
1.2 Aromatase Expression in the Brain
Aromatase is the enzyme that converts androgens to estrogens (P450 aromatase, P450arom, CYP19; (5)). It is abundantly expressed in several areas of the mouse brain, including the hypothalamus, thalamus, preoptic area, olfactory bulb, hippocampus, cerebral cortex, pons, and midbrain. The highest levels of aromatase activity are reported in the hypothalamic region (18, 19). Aromatase expression is also relatively high in the hippocampus of mice (20). In both hippocampal and hypothalamic regions, aromatase mRNA expression is higher in males than females (20, 21). More specifically, the hypothalamic region, which resides within the diencephalons, shows a much higher aromatase expression level in males when compared with female embryonic mice (19). Aromatase activity and mRNA are also expressed in the embryonic striatum and levels tend to increase postnatally in this region as well with no sex differences in aromatase expression (22). Aromatase transcript expression has been detected in the Purkinje cells of rat neonatal cerebellum (23). In the cortex, however, aromatase expression has been reported to be either very low (19) or undetectable (24). Aromatase is also not detectable in the medulla (18).
In general, aromatase is normally found only to be expressed in neurons of the brain, however, it is interesting to note that upon induced brain injury in the mouse, by either kainic acid injections or by a penetrating injury, aromatase becomes expressed in reactive glia in the brain regions affected by the injury (25). Not surprisingly, testosterone treatment increases the aromatase activity within neurons of the hypothalamus, and this is an androgen receptor-mediated event (19, 26). Furthermore, tonic estrogen treatment (in the form of a pellet) was found to upregulate aromatase expression in the hippocampus, whereas 17β-estradiol injections actually decreased aromatase expression in the same region (27). This study emphasizes the importance of selecting the correct method of steroid treatment.
1.3 Estrogen Transport and Metabolism
Following estrogen biosynthesis, which occurs mainly in the ovary in premenopausal women, 17β-estradiol is secreted into the bloodstream where it reversibly binds to sex-hormone-binding globulin, SHB9 (28). It may also bind to albumin in a nonsaturable and nonstoichiometric manner, albeit with a much lower affinity. Approximately, 2-3% of serum estrogen is free and consequently may diffuse into target tissues and henceforth exert further genomic or nongenomic actions (see review (4)).
Estrogens may be metabolized either by sulfation, whereby resulting conjugates are excreted into the bile or urine; or by hydroxylation and subsequent methylation, which leads to the formation of catechol and methoxylated estrogens (see review (4)). Another class of estrogen metabolites are nonpolar fatty esters of estrogens which are named lipoidal estrogens (29). Lipoidal estrogens are synthesized in several tissues in vitro, but reside predominantly in fat. They may also circulate in the blood and can either bind to high density lipoprotein, which is the most common scenario, or may be transferred to low-density lipoprotein by an unknown carrier (29, 30).
2 Estrogen Actions
Estrogens exert their actions via several varying pathways. The classic estrogen activation pathway is through the ligand activated dimerization of nuclear estrogen receptors (estrogen receptor alpha or beta) which mediate transcription of a target gene via its estrogen response element (ERE). Alternatively, the activated estrogen receptor may bind to alternative response elements that differ from EREs and can activate transcription through protein-protein interactions with transcription factors (e.g., AP-1 or SP-1) within the nucleus; these actions are ligand-dependent genomic actions. Estrogens also possess nongenomic ligand-dependent actions, which can occur when estrogen binds to the newly discovered membrane bound estrogen receptors. These are rapid estrogenic actions and lead to activation of the protein kinase cascades. The estrogen receptor may also be activated independently of its ligand. This may occur through the activation of protein-kinase pathways by growth factors (see reviews (17, 31).
2.1 Ligand-Dependant Genomic Activation of Estrogen Receptors
The classic estrogen receptor (ER) activation pathway involves binding of estrogens to nuclear ERα or ERβ, which, upon ligand binding, homodimerize or heterodimerize and bind to a specific response element called estrogen response element (ERE) (see reviews (17, 31)). EREs are expressed in the regulatory regions of estrogen target genes, and the consensus ERE sequence was found from the Xenopus laevis vitellogenin gene with the sequence 5′GGTCAnnnTGACC3′ (32). Very few estrogen inducible genes contain this consensus ERE, but several variants of the sequence that bind estrogen receptors with less affinity have been shown (33). In addition, varying EREs may act in combination to confer estrogen responsiveness (34).
The estrogen-estrogen receptor complex may also bind in the nucleus to nuclear receptor coactivators such as steroid receptor coactivator protein (P160) (35) and p300-cyclic AMP response element binding protein (CBP) (36) or corepressors (37). Estrogens may also exert ERE-independent gene transcription through protein-protein interactions with transcription factor complexes that are in contact with DNA, see review (17). One example of this mechanism is the binding of estrogen receptors to the subunits of activating protein 1 (38). Genes regulated by ER activation of the AP-1 site include ovalbumin (39) IGF-1 (40), collagenase (38) and cyclin D1 (41, 42), and choline acetyltransferase is repressed by ER mediated AP-1 activation (43). ER activated protein-protein interactions include interactions between ER and nuclear factor κβ (44), as well as interactions of ERs with Sp-1 transcription factor (45). Genes activated by the latter include low-density lipoprotein receptor (LDL receptor) (46), c-fos (47) and cyclin D1 (48), whilst nuclear factor κβ along with CCAAT/enhancer binding protein β, are involved in repression of the IL-6 gene (49).
2.2 Ligand-Dependent Non-genomic Activation of Estrogen Receptors
There is compelling evidence that estrogens can rapidly alter neuronal activity within seconds (reviewed in (50)). These rapid actions of estrogen cannot be explained by nuclear activation of ERs but inferred the involvement of nongenomic actions via membrane receptors. Examples of such nongenomic, rapid actions of estrogen include mobilization of intracellular calcium, stimulation of adenylate cyclase activity, cAMP production, and activation of MAPK and PI3 kinase signaling pathways, see review (17).
17β-estradiol has been demonstrated to induce progesterone synthesis in astrocytes through membrane associated ERs and calcium mediated phospholipase C pathway (51). A membrane estrogen receptor mediates intracellular calcium release in astrocytes. Speculation of a membrane-bound estrogen receptor has been circulating for over three decades with reports of outer surface binding sites for estrogen being located in many tissues, including endometrium (52), spermatozoa (53), hepatocytes (54), and mammary gland (55). Several studies have alluded to the possibility that this membrane-bound ER may be a variant membrane-bound form of the classical nuclear ER (see review (56)). Indeed, data from ERα knockout (KO) and ERβKO mice support the role for classical ERs in rapid actions of estrogens. Further, ERα has been shown to be translocated to the plasma membrane through the involvement of two adaptor proteins, Shc and IGF-1, see review (31). Two other nonclassical membrane ERs have been reported thus far. In 2005, G-protein coupled receptor 30 (GPR30) was first reported as an orphan nuclear receptor, which contains binding and signaling characteristics reminiscent of a membrane ER from studies using SKBR3 breast cells (31, 56, 57). However, the role of GPR30 as an ER has been disputed and requires further substantiation (31). A novel plasma membrane-bound ER, containing high affinity saturable estrogen binding sites and located within caveolar-like structures of postnatal but not adult WT and ERKO mice neocortex and uterine, was found to be functionally distinct from the classical ERs (58, 59). Signaling pathways activated by the membrane-bound ERs include calcium flux and ion channels, cAMP, MAPK, PKA, PKC, PI3-K and Src kinase, as well as G-protein coupled receptors and enzyme linked receptors, see reviews (31, 56).
2.3 Ligand-Independent Activation of Estrogen Receptors
A large percentage of nuclear receptors are phosphoproteins, whose functions can be altered by changes in their phosphorylation in the absence of a hormonal ligand (4, 60). Estrogen-independent action of the receptor molecule may occur through phosphorylation of the receptor by protein kinase activators such as growth factors, see review (4). This phosphorylation process occurs mainly at specific serine or tyrosine residues and may be catalyzed by enzymes such as receptor tyrosine kinases and mitogen activated protein kinases (61). Here, the unbound but activated receptor will then exert transcriptional effects (reviewed in (4)). Estrogen independent receptor activation has previously been demonstrated though the actions of dopamine (62), epidermal growth factor, transforming growth factor α (63), insulin or insulin-like growth factor 1 (64), heregulin (65) and cyclic AMP (66).
2.4 Estrogen Receptor Expression in the Brain
In the mouse, ERα and ERβ are abundantly expressed throughout the brain within the cell nuclei of select regions, including the olfactory bulb, cerebral cortex, septum, preoptic area, BnST, amygdala, paraventricular nucleus (PVN), thalamus, ventral tegmental area, substantia nigra, dorsal raphe, locus coeruleus, and cerebellum. Extracellular immunoreactive ER staining has also been detected in the olfactory bulb, CA 3 stratum lucidum and CA1 stratum radiatum of the hippocampus and the cerebellum (67). Interestingly, the expression patterning of estrogen receptors within the brain is similar to aromatase, whereby levels occur prenatally and increase until birth (68). The presence of both aromatase and ER in the brain signifies the fact that estrogens have important roles in brain and behaviors.
3 Models of Estrogen Deficiency
The majority of rodent models used to analyze the physiological effects of estrogen were gonadectomized followed by placebo or 17β-estradiol replacement. A major problem with such studies is that the local brain aromatase may still convert circulating androgens to estrogens, which may “mask” the effects of estrogen withdrawal. Hence, the generation of a complete estrogen-deficient mouse model would uncover more effects of estrogens.
3.1 Generation of the Aromatase Knockout Mouse
The aromatase knockout (ArKO) mouse is a complete estrogen-deficient model. As mentioned previously, the final step in estrogen biosynthesis is catalyzed by aromatase, encoded by the Cyp19a1 gene. Therefore, a disruption to the Cyp19a1 gene would lead to aromatase deficiency and consequently estrogen deficiency. Aromatase knockout mice were generated by the targeted deletion of exon IX of the Cyp19a1 gene (69). Exon IX was selected for targeted disruption because this exon is highly conserved among all the aromatase cDNAs reported to date (70) and computer alignment of the aromatase amino acid sequences indicated that this sequence within exon IX contained several important structural features of the enzyme, including the K and K′ helices, and a number of β-sheet regions (69, 71).
3.2 Phenotypes of the ArKO Mouse
Although no significant morphological abnormalities are seen in the ArKO mice, the uteri of the adult (12-14 weeks) ArKO female are remarkably underdeveloped (69). Interestingly, female ArKO mice develop testicular tissue (Sertoli-like cells) within their ovaries (72). Gonadal and infra-renal fat pad weights of female adult ArKO mice are increased by 50-80% than that of wildtype (WT) controls (76).
The male adult (12-14 weeks) ArKO mice exhibit elevated levels of testosterone and luteinizing hormone, but normal levels of follicle-stimulating hormone. They are fertile with normal testicular morphology at a young age. However, as the mice age, a disruption in spermatogenesis and grossly dysmorphic seminiferous tubules become evident (73). This is accompanied by a decrease in the round spermatid number, which was found so because of an increased apoptosis (73). Of considerable notice is the marked disruption in male sexual behavior observed in the ArKO mice. ArKO males show reduced mounting and decreased intromissions with increased latencies to mount, toward the receptive females, and this phenotype worsened with age (74). In addition to sexual behavior defects, male ArKO mice also showed a loss of aggressive behavior toward the intruder mice when examined using the resident intruder paradigm (75).
Another striking phenotype observed is the increased intra-abdominal adiposity of male ArKO mice when compared to WT controls. This phenotype is a consequence of hyperplasia and hypertrophy of adipocytes. It is also exacerbated with age but can be ameliorated by estradiol replacement (76). In addition, ArKO males present a decrease in lean mass, hypercholesterolemia, hyperleptinemia, and insulin resistance (76). Furthermore, hepatic steatosis or fatty liver is also evident in the male ArKO mice. This male-specific phenotype present pale colored liver which is a result of increased lipid, especially triglyceride, accumulation (77). Estrogen treatment for just 6 weeks was sufficient in blocking and/or reversing the lipid accumulation within the liver (77).
Both male and female ArKO mice develope osteopenia in the lumbar spine, along with an osteoporotic phenotype characterized by significant decreases in trabecular bone volume and thickness (78). In male ArKO mice, only a clear suppression of bone formation has been observed with significant reductions in osteoblastic osteoid and mineralizing surfaces and a 9% reduction in femur size as compared with WT counterparts (79).
3.3 Other ArKO Models
Since the initial generation of the first ArKO mouse in 1998 (69), two more aromatase knockout mouse lines were generated in the laboratories of N. Harada and K. Toda in Japan (80–82). The group from the Toda laboratory generated the ArKO mouse in the same fashion as the Simpson laboratory (69), i.e., disruption of exon IX. Consequently, similar phenotypes were observed in this ArKO mouse line to the original phenotypes observed by Fisher and others (73, 76–78). The group from the Harada laboratory however generated an aromatase knockout mouse by targeting exon I and II of the Cyp19 gene (80). Phenotypes of this knockout mouse line are quite similar to those observed by Fisher et al. (69).
3.4 Estrogen Receptor Alpha Knockout ER a KO Mouse
In order to generate the ERα knockout mouse (83), a neo gene cassette was inserted into the Not1 site within exon 2 of the ER gene, resulting in the disruption of the reading frame.
Externally ERαKO males and females appear normal, however internally females present gross differences, including hypoplastic uteri as well as cystic and hemorrhagic follicles within the ovaries along with a lack of corpa lutea within the ovaries (83). Male ERαKO mice presented testicular atrophy, which is accompanied by decreased spermatogenesis and inactive sperm with an overall sperm count of only 10% that of control levels (83). In addition, dilation of the lumen of seminiferous tubules has been reported to occur due to an impaired reabsorption of luminal fluid in the epididymus (84–86).
All homozygous females are infertile with no signs of lordosis posture or receptiveness to WT males, even upon estrogen priming (83). In the original phenotype of male ERαKO mice, although sexual behavior was impaired, 3 out of 15 pairs produced offspring indicating reduced but not completely abrogated fertility (83). However, since this original report the ERαKO mouse has been backcrossed onto the C57/BL6 strain and subsequently no successful copulatory performance has been demonstrated (83, 84, 87). This stresses the importance of strain differences when analyzing knockout models.
Other phenotypes of the ERαKO mouse include abnormal breast development and bone defects (reviewed in (88)). In addition, female ERαKO mice show increased body weight due to an increase in the white adipose fat in pads of the mammary gland and lateral ventral portion of the body cavity (see review (89)). Only one case of estrogen insensitivity in a human has been reported – an XY male was found to be homozygous for a single point mutation of exon II of the ERα gene resulting in a premature stop codon (see review (89)).
3.5 Estrogen Receptor Beta Knockout ERβKO Mouse
The ERβKO mouse was generated in 1998 by insertion of a neo gene into exon 3 of the ERβ coding gene (90). Early reports indicated no discernable external or histological phenotype of the ERβKO compared to the WT counterparts. However, while females were fertile, they produced smaller litters which were attributed to reduced ovarian efficiency (90). Breast development was reported as normal (90). Young, sexually mature males showed no disruptions in fertility, whereas aged ERβKO males displayed signs of prostate, urethra, and bladder hyperplasia, indicating that growth control of these tissues has been impaired (90).
3.6 Estrogen Receptor Alpha Beta Double Knockout Mouse
In order to study the differential effects of the two ER subtypes, Dupont and colleagues generated their own ERαKO, ERβKO and double estrogen receptor alpha beta double knockout (ERαβKO) models. Although the ERβKO mouse was generated in a similar fashion to (90), the ERαKO mouse was generated this time by targeted disruption of exon 3 with a Cre-lox system (91). Double ERαβKO heterozygous mice (ERα+/− / ERβ+/− ) were obtained from breeding ERα+/− females with ERβ−/− males. These mice were then bred to obtain homozygous ERαβKO mice (91).
When comparing all three receptor knockout mice, the ERαβKO was similar to the ERαKO in terms of male and female infertility and displayed similar hemorrhagic cysts and abnormal antral follicles to the ovaries of female ERαKO mice. An additional phenotype of the ERαβKO was the presence of cells reminiscent of Sertoli cells in the ovaries of female mice. This phenotype is absent in ERαKO and ERβKO, but present in female ArKO mice (91, 92). In male ERαβKO mice, a loss of germ cells in the seminiferous tubules and dilation of tubules was reported in the testis (91).
4 Estrogen-Deficient Mouse Models in the Study of Brain Injury and Ischaemia
While several studies have been conducted on estrogens’ neuroprotective effects in neurodegenerative disease, more recently estrogens have been shown to possess strong neuroprotective properties following brain injury or stroke. Evidence first arose when several studies found that women were less vulnerable to acute brain injuries following cerebral ischemia, hypoxia, and drug-induced toxicity (see review (93)). The incidence of cerebrovascular stroke in premenopausal women is significantly lower than men. However, no such sex differences were observed between postmenopausal women and age-matched men. This suggested a strong case for estrogens in the prevention of stroke and/or traumatic brain injury, and indeed, several studies proved that estrogen treatment diminished the extent of injury (see review (93)). In addition, continued use of estrogens has been demonstrated to reduce the risk of stroke by 50% (see review (94)).
Rat and mouse models of ischemic brain injury and stroke, produced by middle cerebral artery occlusion (MCAO) have shown a higher recovery rate and significantly reduced brain damage following 17β-estradiol treatment; both pharmacological and physiological 17β-estradiol concentrations were effective (see review (95)). This effect was region specific as whilst 17β-estradiol treatment reduced cerebral and hippocampal infarct damage, it had no effect on the striatum following MCAO (95, 96). Indeed, the female ArKO mice showed greater ischemic brain damage after MCAO, when compared to WT controls, confirming the neuroprotective role for estrogen (97).
Neuroprotective effects of estrogen in MCAO animal models have been reported to be mediated via ERα only in the hippocampus, and via activation of both ER subtypes in the subventricular zone (96, 98). More recently it was demonstrated that ERα is induced in the early stages of MCAO injury, whilst ERβ plays a role in the later stages (99). Mechanisms of neuroprotection afforded by estrogen in MCAO models include antiapoptotic regulation of bcl-2 expression, and anti-inflammatory effects via decreasing levels of the inducible isoform of nitric oxide synthase (iNOS) (100, 101).
The ERα subtype has also been to be the predominant receptor in estrogen-mediated neuroprotection from ischemic and stroke induced insults, by comparing ERαKO with WT mice (102, 103). However, there are some discrepancies in the literature. In fact, some studies have found that the use of ERαKO and ERβKO models has actually led to the discovery that neither ER subtypes may be involved in estrogen-regulated neuroprotective mechanisms, and rather the proposed third membrane-bound estrogen receptor is responsible. Indeed the membrane-bound or ER-X receptor is believed to be responsible for estrogen-regulated activation of the mitogen-activated protein (MAP) kinase cascade (104, 105). Furthermore, the ER-X receptor, which is believed to be situated within the caveolar-like microdomains of postnatal but not adult WT and ERKO neocortex and uterine plasma membranes, is reexpressed in the adult brain following ischemic stroke injury (59). Such studies represent great prospects in rapid acting neuroprotective actions of estrogens in the therapeutic setting.
5 Estrogen-Deficient Mouse Models in the Study of Neurodegenerative Diseases
The neuroprotective effects of estrogens are demonstrated again in the aromatase knockout (ArKO) – the complete estrogen-deficient mouse model. Recently, we reported the presence of age-exacerbated apoptosis in layers II and III pyramidal neurons of the female ArKO frontal cortex, with concomitant decreased expression of bcl-2 related antiapoptotic genes and increased expression of the pro-apoptotic gene TRADD (106). These phenotypes could be reversed by 3-week estrogen replacement. Without estrogen replacement, by 12-15 months of age, the female ArKO mice have 30% less neurons and lower dry brain weight (106). Such neuronal death was not detected in the WT littermates or male ArKO mice. Instead, apoptosis occurred in the dopaminergic neurons in the arcuate nucleus and the MPO of 1-year-old male ArKO mice, which may be a consequence of the activation of the death receptor pathway (107). This neuronal death could be prevented by 6 weeks of estrogen replacement (107). Furthermore, apoptosis in the arcuate nucleus and MPO could be prevented by ERα selective agonist and ERβ agonist, respectively (108, 109). The basis of the sexual dimorphism of the neuronal death phenotypes remains to be elucidated.
Depletion of dopaminergic synthesizing neurons within the substantia nigra pars compacta is the main characteristic of Parkinson’s disease, a disorder which leads to progressive motor dysfunction. Parkinson’s disease is more prevalent in men than in women and, in addition, symptoms evolve quicker and men are less responsive to levodopa treatment than women (see review (110)). These differences may be attributed to the level of steroid hormones such as testosterone and local estrogen levels. Whilst some studies have demonstrated that estrogens improve motor disability in post-menopausal women with Parkinson’s disease (111), others have found no effects of estrogen therapy (112, 113). Besides dopaminergic cell loss, Parkinson’s degeneration also involves oxidative stress, excitotoxicity, and metabolic challenges, and estrogen may exert protective properties through the disruption of many of the degenerative processes (see review (110)).
Estrogens have also been reported to decrease the incidence and delay the onset of Alzheimer’s disease (AD), but this is a point of great debate. AD is more prevalent in women than in men and its incidence increases with age, rising from 1.2 per 1,000 at age 65 to 63.5 per 1,000 by age 90 (see review (114)). This age-related increase coincides with menopause in women, and therefore suggests a role for steroid hormones in protection against AD (see review (114)). Several earlier small, short-term trials on hormone replacement therapy suggested that female AD patients receiving hormone replacement therapy (including estrogen supplements) have milder symptoms of dementia when compared with female AD patients not using hormone replacement therapy (115, 116). However, larger later trials involving longer treatment periods reported no difference in the cognitive or functional outcomes between female AD patients with or without estrogen treatment (see review (114)). Several explanations have been proposed for such discrepancies – the type of estrogen replacements used, and/or the age at which estrogen therapy was initiated may be causative factors. Indeed, studies have shown that early in menopause, when neurons are still in a healthy state, estrogen therapy can be quite effective, but once the disease has progressed and is manifested in the neurons, estrogen treatment appears to be ineffective (see review (94)).
Several studies have found that pretreatment with 17β-estradiol prior to exposure to amyloid β peptide, a neurotoxic peptide found in AD, protected neurons in a significant manner (117–122). However, whilst some explain estrogens’ neuroprotective actions here by antioxidant effects (118, 121), others describe the neuroprotection afforded by estrogen in amyloid β induced toxicity to be an ER-mediated antiapoptotic effect (119, 120, 122). Estradiol pretreatment has also been shown to be efficient in neuroprotection against the cytotoxic effects of 1-Methyl-4-phenyl pyridium (MPP+) using neuronal PC12 cells in a cell culture model of Parkinson’s disease (123). In addition, 17β-estradiol treatment restored the dopamine transporter protein expression levels in MPP+ treated cultures to control levels (123).
Taken together, these in vitro studies demonstrate that 17β-estradiol has strong neuroprotective properties. However, several key factors need to be taken into consideration when analyzing these neuroprotective actions. Firstly, the majority of these studies only found significant effects with pretreatment of 17β-estradiol before the onset of neuronal toxicity. Secondly, the dosage of 17β-estradiol administered seems to play a role in the effectiveness of the treatment, and the mechanism of action (i.e., rapid membrane effects, or slower genomic effects). Finally, the specific brain region in question may react differently to 17β-estradiol treatment.
Mouse models of neurodegenerative disorders, such as Parkinson’s disease and Alzheimer’s disease, have also benefitted with 17β-estradiol treatment. Parkinson’s disease mouse models have been created by inducing lesions within the striatal region via injection of 1-Methyl-4-phenyl-1-1, 2, 3, 6-tetrahydropyridine (MPTP). Sex differences in the neurotoxic effects of lesioning have been shown, with males displaying more dramatic dopaminergic cell loss than females, indicating the involvement of sex steroid hormones (see review (110)). Indeed, 17β-estradiol treatment could reduce the increased level of glial fibrillary acid protein (GFAP) caused by MPTP induced lesions (see review (110)). Furthermore, 17β-estradiol treatment has demonstrated rapid dose-dependent increases in dopaminergic neuron activity within the striatal region of ovariectomized rats (see review (110)). In concurrences to these observations, ArKO females showed significantly greater vulnerability to MPTP-induced nigrostriatal damage than their WT counterparts. In addition, functional integrity of SNpc tyrosine hydroxylase-positive neurons and dopamine transporter innervation of the caudate-putamen of the ArKO were impaired (124).
Several discrepancies in the literature have arisen over the protective effects of estrogen found in mouse models replicating AD. The extracellular deposition of amyloid peptides in plaques and neurofibrillary tangles are the two characteristics in the pathology of AD (125). Mutant amyloid β protein precursor transgenic mice, ovariectomized and treated with placebo, 17α- or 17β-estradiol showed decreased amyloid β levels by 27% and 38% in 17β and 17α estradiol treated mice respectively (125). Furthermore, this amyloid β lowering effect was replicated with lower physiological levels of 17α and 17β-estradiol applied in the drinking water, and thus presents a strong case for the beneficial effects of estrogen on reducing amyloid β plaques (125). Furthermore, a separate study found that the gene-encoding transthyretin, which has been reported to scavenge amyloid β peptides and reduce the amyloid plaque formation, was found to be increased in ovariectomized mice treated with 17β-estradiol when compared to placebo-treated ovariectomized mice (126). On the other hand, other studies using amyloid precursor protein transgenic mice have reported no effects of ovariectomy or estrogen replacement on amyloid beta deposition in the hippocampus and neocortex (127, 128). However, when the APP23, AD mouse model was cross bred with the ArKO model, their estrogen-deficient APP23 offspring did present an earlier onset of AD symptoms, accompanied by an increased β amyloid peptide deposition and increased amyloid β production (129). Cell cultures from the brains of these offspring also showed a marked impairment in amyloid β clearance and degradation (129).
Therefore, further studies combining Parkinson’s disease or Alzheimer’s disease models with ArKO or ERKO mice will certainly aid our understanding of the neuroprotective role of estrogens in neurodegenerative disease.
A more recent report noted differential effects of the two ER subtypes on the expression of apolipoprotein E as a risk factor for Alzheimer’s disease, indicating that past differences may be due to differences in the ER subtype expression within the specific brain regions analyzed (130). These in vivo studies certainly strengthen in vitro experiments demonstrating the strong neuroprotective properties of estrogen.
6 Estrogen-Deficient Mouse Models in the Study of Cognitive Functions
Estrogens have been demonstrated to enhance some aspects of cognitive function in human and animal models although the demonstrated effects are often modest and inconsistent across studies (see review (131)). Conflicting results have also been reported in the estrogen deficient models.
Both male and female ArKO mice performed significantly worse than WT controls in tests for short term memory by use of the Y-maze test, thus revealing an important role for estrogen in the memory process (132). On the other hand, during the Morris Watermaze test (which test the spatial learning ability of the rodents), the estrogen-deficient female ArKO mice performed equally well, if not better than, as the WT counterparts (133). Interestingly, WT females treated with estradiol benzoate failed to learn this spatial discrimination task (134), whereas WT males and ERαKO of both sexes, regardless of treatment, performed equally well. In addition, significantly higher hippocampal levels of the N-methyl-D-aspartate (NMDA) receptor subunit NR2B transcript has been demonstrated by real-time PCR in female ArKO mouse brains as compared to WT (133). NR2B is involved in the Long-term Potentiation of memory formation and learning, thus indicating that estrogens may have an inhibitory effect on spatial learning (via ERα) in females.
7 Estrogen-Deficient Mouse Models in the Study of Schizophrenia, Depression and Compulsive Behavior
Prepulse inhibition is a model of sensorimotor gating mechanisms necessary for normal sensory information processing in the brain (135). Deficiencies in prepulse inhibition have been found in patients with schizophrenia (136), Tourette’s syndrome (137), obessive-compulsive disorder (138). It has also been reported that women show less inhibition than men, especially when weaker prepulse was used (139).
Interestingly, male ArKO, but not female ArKO mice, showed an age-related reduction in prepulse inhibition, and significantly greater amphetamine-induced hyperactivity, thus indicating a neuroprotective role for estrogen, particularly in the aging brain, in mechanisms involved in prepulse inhibition (PPI) and locomotor activity regulation (140). This led the authors to postulate a role for estrogen in the regulation of dopaminergic and/or serotonergic activity in the male (140). Indeed, further studies showed that male ArKO mice show a greater response to serotonin receptor, 5-HT1A receptor stimulation on PPI behavior experiments (141). In fact, clinical trials of treatment with 17β-estradiol, for women diagnosed with schizophrenia has demonstrated that estradiol may have antipsychotic properties, or act as a catalyst for neuroleptic responsiveness (see review (110)).
Although female ArKO mice do not show PPI deficit, they display decreased active behaviors, such as struggling and swimming, and increased passive behavior, such as floating during a forced swim test situation. Such behaviors are indicative of depressive-like symptoms, and these behaviors are accompanied by a concomitant decrease in the serotonergic activity within the hippocampus of ArKO female when compared to WT controls. Estrogen replacement can not reverse these phenotypes, thus suggesting that they are the consequence of the absence of estrogens during development (142). By contrast, male ArKO mice do not show such depressive-like symptomology (143). This seemed to mirror the observation that more women suffer from depression than men, across all age groups in the United States (see review (144)). The anti-depressive effects of estrogens have also been demonstrated by administering SERMS (selective estrogen receptor modulators) to ovariectomized rodents. Administration of ERβ-selective SERMs to the hippocampus, but not the ventral tegmental area, decrease anxiety and depressive behavior (145). When the ERβ has been disrupted, as in the ERβKO, the anti-depressive effects of 17β-estradiol are not detectable (146).
We have recently reported that by 6 months of age, male, but not female, ArKO mice presented compulsive behavior such as grooming and wheel-running (108, 109). They did not present general hyperactivity, but lower ambulatory activity instead. The hypothalamus is involved in grooming (147), with the medial preoptic area regulating both grooming (148) and wheel-running behaviors (149, 150). Indeed, the protein expression levels of the catecholamine degrading enzyme, catechol-O-methyltransferase (COMT), are significantly lowered in the male ArKO hypothalamus (including the medial preoptic area), but not in the frontal cortex (108, 109). COMT is involved in the metabolism of dopamine, and hence a possible consequence may be an increased dopamine level in the hypothalamus leading to the development of excessive grooming and wheel-running activities in the male ArKO. By contrast, no such behavioral phenotypes have been observed in the female ArKO mice, which are paralleled by the normal presentation of hypothalamic COMT levels (108, 109). In addition, no such compulsive behaviors have been reported in the ERKO animals.
As estrogen is required during neuronal development, we cannot rule out the possibility that the compulsive-related phenotype presented in the male ArKO mice is a result of estrogen deficiency during development. Nonetheless, just 3 weeks of 17β-estradiol replacement did restore the COMT levels in the ArKO hypothalamus with concomitant amelioration of the compulsive behavior (108, 109). Thus, decreased estrogen levels correlate with a specific decrease in the hypothalamic COMT expression and development of compulsive behavior (i.e., grooming and wheel-running in male mice).
The first line of treatment for OCD is selective serotonin reuptake inhibitors (SSRIs). A proportion of OCD patients (40-60%) do not respond to SSRI-monotherapy (151, 152) but considerable symptomatic improvement may be seen when prescribed with a dopamineragic antagonist (153). This implies that both the dopaminergic and serotonergic systems mediate OCD. The involvement of the dopaminergic system in OCD is further supported by studies showing that dopamine agonists induce OCD behaviors in rats (154, 155). Hormonal influences on OCD have also been implicated with female patients reporting that symptoms worsen during premenstrual/menstrual periods (156) or start after menopause or within 1 month of childbirth (157) – suggesting that estrogen withdrawal may be involved. Furthermore, male OCD patients have symptoms at a younger age and with worse outcomes (158, 159).
8 Estrogen-Deficient Mouse Models in the Study of Sexual Behavior
Loss of sexual behavior was first reported in the exon 1 and 2 disrupted male ArKO mouse in 1998 (80). This report was confirmed when exon IX-disrupted ArKO males were reported to show reduced mounting and decreased intromissions with increased latencies to mount, toward receptive females, and this phenotype worsened with age (74). Further exploration of the exons 1 and 2-disrupted ArKO model found that along with decreased coital behavior, the male ArKO mice showed reduced aggression toward WT male intruders (160), and in addition, impairments in olfactory investigations (161). Moreover, while estradiol benzoate and dihydrotestoersterone proprionate (DHTP) treatment restored sexual behaviors to WT levels, these treatments had no effect on the olfactory investigation (161). This effect of estradiol upon sexual behavior seems to be a rapid effect, as a single injection of estradiol given to a male ArKO was able to activate sexual behavior within 15 min (162). Female ArKO mice have also been reported to display impairments in sexual receptivity, as well as deficits in olfactory investigation (163). This deficit in olfactory investigation may be due to alterations in the processing of male odors, stemming from the ventromedial hypothalamus (164).
Sexual behavior studies on ERKO knockout models revealed that male ERαKO mice show reduced levels of intromission and ejaculation, while mounting behaviors were unaffected (165). In contrast, male ERβKO mice display all components of sexual behavior, with no apparent effects of genotypes (166). However, the double ERαβKO do not show any components of sexual behavior including mounting behavior (167). The sexual behavior phenotype of the ERαβKO is in fact similar to the male ArKO sexual behavior phenotype. Female ERαKO mice are infertile and do not appear to engage in reproductive behaviors for two reasons: one is that the stud male believes that the ERαKO females are in fact intruder males and proceed to attack the females rather than mount; and secondly, even after treatment with strong cutaneous stimuli, which usually promote lordosis behavior, the ERαKO female does not display any sexual behavior characteristics such as immobilization or vertebral dorsiflexion (see review (168)). This was further confirmed by the work of Rissman and colleagues who found by use of the ERαKO model that ERα is indeed required for sexual receptivity but not essential for female attractivity (169). However, male ERαKO mice do not exhibit the same social preferences for female mice as do their WT littermates (170). Other behavioral phenotypes of the male ERαKO include a loss of aggressive behavior and female-typical rather than male-typical emotional responses in open field tests see review (168). Male ERβKO mice, on the other hand, display loss of visuospatial learning, and further a role for ERβ has been suggested in the defeminization of sexual behavior (see review (171)).
9 Summary
In summary, estrogens may act through several different ER-dependant and ER-independent pathways to exert neuroprotective actions in the brain and the actions of estrogens appear to be heavily dependent upon the dosage of estradiol administered, and on the region in which the brain assault has occurred. Use of the aromatase knockout mouse to investigate roles of estrogens in the mammalian brain ensures that the brain is in a complete state of estrogen deficiency, and therefore is an excellent model to work with. In addition, estrogen receptor knockout mice enable us to decipher the exact roles of each specific estrogen receptor subtype in modulating the neuroprotective effects of estrogens. In future, the creation of double mutants, such as crossing estrogen-deficient models with a neurodegenerative disease or brain injury mouse model (e.g., ArKO crossed with APP23), is an excellent tool for studying the effects of estrogens on neuroprotection.
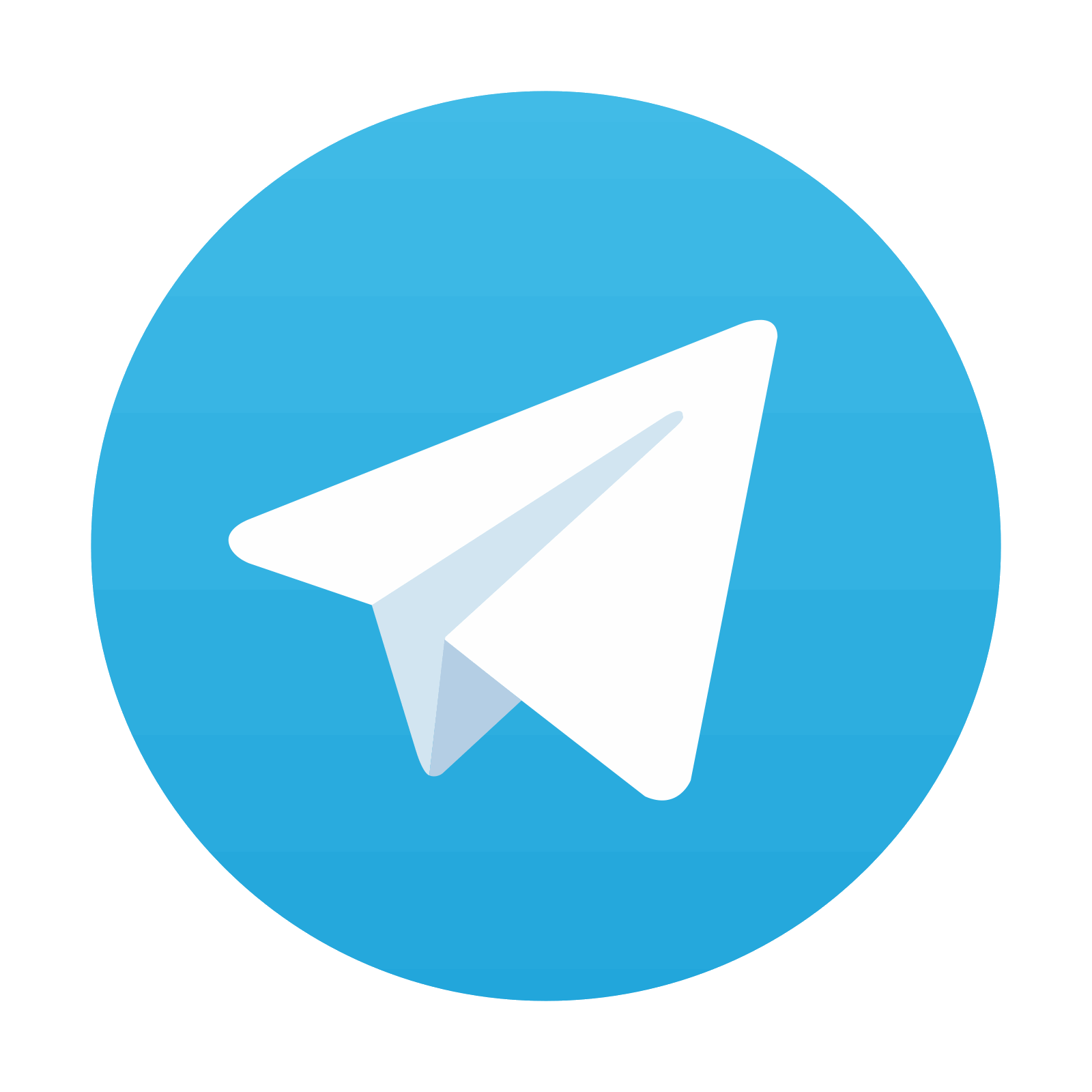
Stay updated, free articles. Join our Telegram channel
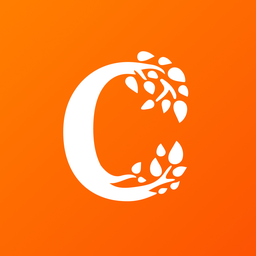
Full access? Get Clinical Tree
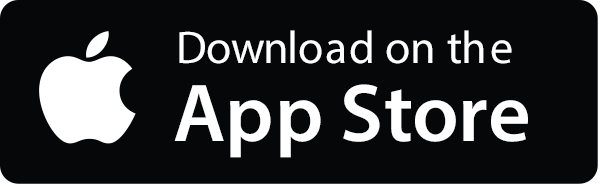
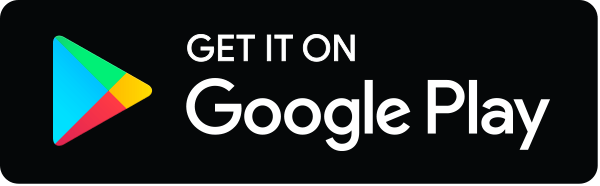