Abstract
Neuromuscular disorders may cause dramatic disability in the neonatal period. The dominant features of these disorders are muscle weakness and hypotonia. In this context, we consider as neuromuscular disorders those that predominantly involve the motor system, from its origins in the cerebral cortex to its termination in the muscle. In this chapter, the motor system is described in terms of its anatomical and physiological organization; special additional emphasis is placed on the development and the biochemical features of muscle. Also, the evaluation of disorders of the motor system in the newborn and the diagnostic studies used in their diagnostic investigation are reviewed in detail.
Keywords
neonatal, neuromuscular, hypotonia, evaluation, electromyography (EMG), muscle biopsy, muscle enzymes, genetic testing
Neuromuscular disorders may cause dramatic disability in the neonatal period. The dominant features of these disorders are muscle weakness and hypotonia. In this context, neuromuscular disorders are considered those that predominantly involve the motor system, from its origins in the cerebral cortex to its termination in the muscle.
This chapter and the next three chapters are concerned with neuromuscular disorders. In this chapter, the motor system is described in terms of its anatomical and physiological organization; special additional emphasis is placed on the development and biochemical features of muscle. Also, the evaluation of disorders of the motor system in the newborn and the diagnostic studies used in their diagnostic investigation are reviewed in detail.
Motor System
The control of movement and tone in the human nervous system is highly complex, and there are major lacunae in our understanding of this control in the neonatal period. Nevertheless it is reasonable to expect that the anatomical systems critical for the control of movement and tone in the mature nervous system are operative, although undoubtedly to varying extents, in the immature nervous system. In the following discussion, the major components of the central and peripheral nervous systems that are important for the control of movement and tone are briefly reviewed. The discussion is organized in the framework used in the next three chapters for the categorization of diseases that disturb muscle power and tone in the human infant.
Levels Above the Lower Motor Neuron
Control of muscle power and tone begins in the central nervous system at levels above the lower motor neuron ( Box 30.1 ). This control is mediated in large part by the major motor efferent system—the corticospinal and corticobulbar tracts—often termed the pyramidal system because most of these tracts originate in the pyramidal cells of the motor cortex of the cerebrum. Also important in control, primarily through effects on cerebral cortical motor centers, are the basal ganglia and cerebellum. Certain other descending tracts that have an impact on the lower motor neurons involved in muscle power and tone are the rubrospinal, reticulospinal, and vestibulospinal tracts, sometimes collectively referred to as the bulbospinal tracts .
Levels Above the Lower Motor Neuron
Corticospinal-corticobulbar tracts
Basal ganglia
Cerebellum
Other components (“bulbospinal”)
Rubrospinal tracts
Reticulospinal tracts
Vestibulospinal tracts
Lower Motor Neuron
Cranial nerve motor nuclei
Anterior horn cells
Peripheral Nerve
Neuromuscular Junction
Presynaptic
Postsynaptic
Muscle
Corticospinal and Corticobulbar Tracts
The corticospinal tract is the major efferent system concerned with the movement of the axial and appendicular musculature; the corticobulbar tract is concerned with the movement of muscles innervated by the cranial nerves. The origin of this system in the mature subhuman primate is principally from pyramidal cells, with the following distributions: motor cortex, 31%; premotor cortex, 29%; and parietal lobe, 40%. The topographic representation of the homunculus on the contralateral cerebral cortex (see Fig. 18.9 ) provides an estimate of the somatotopic origin of these fibers. This system descends through the posterior limb of the internal capsule, the cerebral peduncles, and the pontine tegmentum; it decussates in the ventral medulla and then descends in the lateral column of the spinal cord. A small portion of the system does not decussate and descends uncrossed in the anterior column of the spinal cord. The corticospinal tract subserves refined volitional movements, although its precise contribution to movement in the human newborn is not entirely known.
Basal Ganglia
The system of basal ganglia, sometimes categorized by the less precise term extrapyramidal system , principally consists of five major nuclear masses: caudate, putamen, globus pallidus, subthalamic nucleus, and substantia nigra. These nuclei do not project to the lower motor neuron directly but rather influence muscle power and tone primarily by effects on the corticospinal system. The major afferent centers are the caudate and putamen (the corpus striatum), and the major efferent center is the globus pallidus. Output from the globus pallidus is relayed to the cortical motor neurons principally by way of the thalamus.
Cerebellum
The cerebellum is a complex system of neurons concerned with the coordination of somatic motor activity, regulation of muscle tone, and mechanisms that influence and maintain posture and equilibrium. Afferent connections are derived from muscle and tendon stretch receptors and the visual, auditory, vestibular, and somesthetic sensory systems; these connections are conveyed principally through the inferior and middle cerebellar peduncles in the brain stem. Efferent connections are conveyed principally through the superior cerebellar peduncle to the red nucleus (and then to the rubrospinal tract), the vestibular nuclei (and then to the vestibulospinal tract), and the thalamus (and then to cerebral cortical motor neurons). Thus the control of movement and tone by the cerebellum is ultimately by way of other motor systems. The hypotonia observed in cerebellar disease may be mediated primarily by decreased muscle fusimotor activity.
Other Components
The other major components of the motor system include the rubrospinal, reticulospinal, and vestibulospinal tracts, sometimes collectively known as the bulbospinal tracts. The nerve fibers of these tracts, unlike most other fibers of the motor system, are myelinated in the third trimester and may play a particularly important role in the control of movement and tone in both the premature and full-term newborn .
Rubrospinal Tract.
The rubrospinal tract originates in the red nucleus of the midbrain, decussates, and then descends in the lateral aspect of the spinal cord. Major afferents are from the cerebellar and cerebral cortices, and the rubrospinal tract projects to nuclei in the brain stem and cerebellum before reaching the spinal cord. The most important function of the rubrospinal tract is the control of muscle tone in flexor muscle groups. It is tempting to speculate that this system is particularly important in the term newborn because of the impressive flexor tone in the limbs (see Chapter 9 ).
Reticulospinal Tracts.
Reticulospinal tracts emanate from neurons of the reticular formation in the pontine and medullary tegmentum and descend in the anterior aspect of the spinal cord. Afferents are derived from all sensory systems and the cerebral cortex. This system has major functional effects on muscle activity and tone, principally through action on the gamma motor neurons in the anterior horn of the spinal cord, which innervate the contractile portions of the muscle spindle. Indeed, the reticulospinal system presumably mediates the impressive changes in tone observed in infants according to their level of alertness.
Vestibulospinal Tract.
The vestibulospinal tract arises from the lateral vestibular nucleus and descends in the anterolateral aspect of the spinal cord. Afferents are derived primarily from the labyrinth (and vestibular portion of the eighth nerve) and the cerebellum. An increase in extensor muscle tone is observed with stimulation of the lateral vestibular nucleus. This system may play a role in the newborn in the mediation of reflex activity associated with vestibular input and extensor muscle activity (e.g., tonic neck and Moro reflexes).
Lower Motor Neuron
The suprasegmental influences just described play on the final common pathway of the motor system, the motor unit. The term motor unit refers to the lower motor neuron (i.e., anterior horn cell or brain stem neuron of the cranial nerve nucleus ), the peripheral nerve (or cranial nerve), the neuromuscular junction, and the innervated muscle.
In the spinal cord, anterior horn cells are arranged so that neurons subserving the function of extensor muscles are located ventrally, those subserving flexor muscles are located dorsally, those subserving proximal muscles are located laterally, and those subserving distal muscles are located medially. The two major types of efferent neurons are the predominant large cells that innervate striated muscle and the less abundant small cells that innervate the fibers of the muscle spindle, the stretch receptors. The latter are important in determining the activity of stretch (“tendon”) reflexes and receive input from the aforementioned suprasegmental tracts.
Peripheral Nerve
The large anterior horn cells, concerned with the innervation of skeletal muscle, exit through the anterior roots to the peripheral nerve. The nerve fibers conduct the nerve impulse with a velocity directly proportional to their diameter and the size of their myelin sheaths. (In addition to transmission of the nerve impulse, these fibers also transport a variety of compounds, including enzymes, neurotransmitters, organelles, and nutrient materials, to the distal aspect of the fiber.) The terminal aspect of the nerve fiber ramifies into a variable number of smaller fibers that form motor endplates at the neuromuscular junction. The axon of one motor nerve supplies a variable number of skeletal muscle fibers. In the larger muscles involved in postural control, a single anterior horn cell may provide motor endplates to more than 100 muscle fibers. In smaller muscles (e.g., of the thumb) concerned with highly skilled movement, a single anterior horn cell provides endplates for only a few fibers.
Neuromuscular Junction
The neuromuscular junction contains the terminal nerve branch with its specialized presynaptic ending , which lies in a specialized trough of the postsynaptic muscle plasma membrane (sarcolemma). A synaptic cleft separates the two membranes. When a nerve impulse arrives at the presynaptic site, calcium enters the presynaptic axoplasm and causes the release of vesicles of acetylcholine. The neurotransmitter then diffuses across the synaptic cleft, binds with a specific postsynaptic receptor on the sarcolemma, and alters the permeability of the muscle membrane. Depolarization and a muscle action potential result if enough receptors are activated. This electrical signal is transmitted along the muscle membrane and then internally by a system of invaginations of the sarcolemma to provoke the events leading to muscle contraction. The coupling of excitation and contraction is discussed in more detail subsequently.
Muscle
Development
The chronology and major features of the development of skeletal muscle in the human are summarized in Table 30.1 . These features provide information of value in interpreting the pathological significance of specific findings of the muscle biopsy in the newborn and in establishing anatomical correlates for certain developmental changes in muscle function (see subsequent discussion).
DEVELOPMENTAL STAGE | TIME (WEEK OF GESTATION) | MAJOR DEVELOPMENTAL EVENTS |
---|---|---|
Premyoblastic | 0–5 | Differentiation of mesenchymal cells to myoblasts |
Myoblastic | 5–8 | Proliferation of myoblasts |
Myotubular | 8–15 | Formation of syncytium with central nuclei, myofibrils, sarcotubular system, and early endplates |
Myocyte | 15–20 | Movement of nuclei to periphery |
Continued synthesis of myofibrils | ||
Early histochemical differentiation | 20–24 | Differentiation of fiber types I and II; type II fibers predominate |
Intermediate histochemical differentiation | 24–34 | Increase in size of type II fibers |
Late histochemical differentiation | 34–38 | Development of equal numbers of fiber types I and II due to marked increase in small type I fibers |
Mature myocyte | >38 | Increase in size of all muscle fibers |
Premyoblastic Stage.
The premyoblastic stage, occurring in the first 5 weeks of gestation, is characterized principally by the differentiation of primitive mesenchymal cells to myoblasts.
Myoblastic Stage.
The myoblastic stage, which follows in the next 3 to 8 weeks, is dominated by the active proliferation and migration of myoblasts along programmed pathways in synchrony with neural crest migration. Synthesis of the contractile proteins begins at this stage.
Myotubular Stage.
During the myotubular stage, from approximately 8 to 15 weeks of gestation, myoblasts fuse to form the syncytium characteristic of human skeletal muscle. Nuclei are located centrally, unlike the peripheral location of mature muscle. Myofilaments of the contractile proteins, actin and myosin, develop the longitudinal organization necessary for the formation of myofibrils. The sarcotubular system, the invaginations of the sarcolemma so important in excitation-contraction coupling, is formed. Axonal terminals contact muscle at 9 to 11 weeks, and motor endplates begin to appear at 14 weeks of gestation. The genes myogenin and myomaker are the primary mediators of myoblast fusion; myomaker is known as a late membrane activator of that process.
Myocytic Stage.
The myocytic stage, occurring from 15 to 20 weeks, is characterized by migration of nuclei to the periphery of the myotube. Active synthesis of myofibrils continues at this stage.
Early Histochemical Differentiation.
In the stage of early histochemical differentiation, during the period from 20 to 24 weeks, approximately 5% to 10% of fibers develop prominent quantities of oxidative enzymes and correspond to type I fibers; these are distinctly large fibers and are called Wohlfart b-fibers ( Fig. 30.1 ). The remaining, smaller fibers, designated Wohlfart a-fibers or type II because of their adenosine triphosphatase (ATPase) concentration, predominate ( Fig. 30.2 ). These small fibers are relatively undifferentiated and categorized as type IIc fibers, to be distinguished from the differentiated type IIa and IIb fibers that develop in the ensuing weeks and months.


Intermediate Histochemical Differentiation.
In the stage of intermediate histochemical differentiation, between 24 and 34 weeks of gestation, the predominant change is a modest increase in size of type II fibers. No significant changes in type I fiber size occur during this period.
Late Histochemical Differentiation.
In the stage of late histochemical differentiation, during a relatively brief period from 34 to 38 weeks, a dramatic change occurs. Small type I fibers appear in large numbers (see Fig. 30.2 ). Near the end of this stage, almost equal numbers of the two muscle fiber types are observed.
Mature Myocytic Stage.
Subsequent development (i.e., the mature myocytic stage), after 38 weeks, is characterized particularly by the increasing size of individual muscle fibers ( Fig. 30.3 ). Indeed, with the continued synthesis of myofibrillar components, the mean diameter of muscle fibers increases approximately fourfold from term (15 µm) to 13 years of age (65 µm).

Importance of Motor Innervation.
The factors governing the developmental sequence of myogenesis are not entirely understood, although motor innervation likely plays an important role. Denervation of the soleus muscle in the newborn rat, when myogenesis is at the myotubular stage, results in a severe maturational delay , with persistence of myotubes and the failure of histochemical differentiation. The role of the motor neuron in the determination of muscle fiber type has been demonstrated by cross-innervation and reinnervation after denervation experiments. Thus muscle fiber type can be changed according to the motor innervation. In addition to its roles in differentiation, motor innervation also plays an important trophic role in that disturbances of motor innervation also result in the defective growth of affected fibers.
Contractile Elements
The major contractile elements of skeletal muscle are the longitudinally oriented filaments, two sets of which can be recognized: thick and thin. These filaments are arranged in repeating units, imparting its striated appearance to the muscle cell. The two sets of filaments in each repeating unit become cross-linked only on excitation; contraction of muscle then is effected by a relative sliding motion of the cross-linked filaments.
The major myofibrillar proteins that make up the thin and thick filaments are shown in Table 30.2 . The principal protein of the thin filament is actin and that of the thick filament is myosin. The interaction of these filamentous proteins is regulated by proteins in the thin filaments, the tropomyosin-troponin complex. Troponin itself is a complex of three proteins, one of which binds calcium and, in so doing, allows tropomyosin to change its position within the thin filament to permit myosin to combine with actin, which then leads to contraction.
PROTEIN | LOCATION | FUNCTION |
---|---|---|
Myosin | Thick filament | Contraction |
Actin | Thin filament | Contraction |
Tropomyosin, troponin | Thin filament | Regulation of actin-myosin interaction; provision of calcium requirement |
Excitation and Contraction Coupling
Major insight into the coupling of muscle excitation and contraction has been gained. The major events in this fascinating sequence are depicted in Box 30.2 . The initial event is the release of the prepackaged vesicles of acetylcholine from the presynaptic nerve ending, provoked by the arrival of the nerve action potential and the subsequent influx of calcium. Acetylcholine traverses the synaptic cleft and binds to a specific postsynaptic receptor on the sarcolemma, thus initiating depolarization of the muscle membrane. Propagation of depolarization occurs along the sarcolemma as well as interiorly. This penetration of depolarization into the interior of the cell occurs by way of the transverse tubules, which are continuous with the outer membrane. In close contact with these tubules is the sarcoplasmic reticulum, membranous sacs rich in calcium. With the inward propagation of depolarization, calcium is released from the sarcoplasmic reticulum and binds with a peptide of the troponin complex. This allows the movement of tropomyosin within the thin filament alluded to previously, the result of which is the interaction of actin and myosin necessary for contraction. Contraction results when actin combines with myosin, thereby stimulating myosin ATPase activity and hydrolysis of adenosine triphosphate (ATP). This occurrence is critical because ATP serves to dissociate actin-myosin cross-links. With a decrease in ATP, the cross-links occur, and the relative sliding motion of the filaments causes contraction. Relaxation is associated with the return of calcium into the sarcoplasmic reticulum, an event mediated by an ATP-dependent calcium pump.
The motor nerve action potential causes the release of acetylcholine at the neuromuscular junction.
Acetylcholine initiates depolarization of the muscle cell membrane.
Depolarization penetrates into the interior of the muscle cell through the transverse tubules.
The adjacent sarcoplasmic reticulum releases calcium.
Calcium binds to the troponin component, thus allowing tropomyosin to change position within the thin filament and, in turn, actin to interact with myosin.
The interaction of actin and myosin causes sliding movement of the thin and thick filaments and contraction.
Biochemical Features
Energy is required for the work of muscle contraction; thus the major task of muscle metabolism is to generate ATP. However, the synthesis of structural, contractile, and other components is obviously also of great importance, particularly during myogenesis. The major metabolic mechanisms for energy production in muscle differ primarily according to the functional requirements of a given fiber.
Two essential fiber types can be recognized by histochemical criteria ( Table 30.3 ). Type I fibers are generally concerned with slow, sustained activities and are rich in oxidative enzymes. Type II fibers are generally concerned with rapid bursts of activity and are rich in glycogenolytic (phosphorylase) and glycolytic enzymes. Although the metabolic and physiological correlates are complex, it appears that fibers involved in rapid bursts of activity derive their ATP principally from carbohydrate metabolism (i.e., glycogenolysis and glycolysis). When more sustained activity is needed, glycogen stores are not adequate and another source of ATP is needed; in largest part, this source is lipid (i.e., fatty acid).
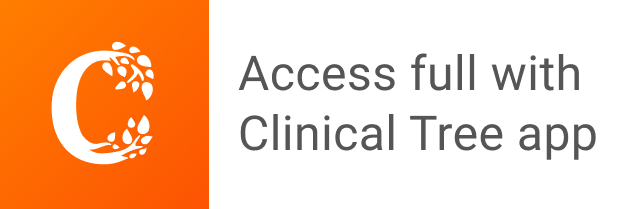