Objectives
- 1.
Compare and contrast the mechanisms of excitation-contraction coupling in skeletal, cardiac, and smooth muscle cells.
- 2.
For skeletal muscle, describe:
- a.
How movement of a voltage sensor couples sarcolemmal depolarization to the opening of SR Ca 2+ release channels.
- b.
The source of the Ca 2+ required for the activation of contraction.
- c.
What happens to the Ca 2+ to effect relaxation.
- a.
- 3.
Describe how cardiac muscle is activated by Ca 2+ ions in the process known as Ca 2+ -induced Ca 2+ release (CICR) .
- 4.
Describe the roles of SERCA, the Na + /Ca 2+ exchanger (NCX), and the plasma membrane Ca 2+ pump (PMCA) in the relaxation of cardiac muscle.
- 5.
Explain how diverse smooth muscles can be activated by depolarization or by agonists through a process known as pharmacomechanical coupling.
- 6.
Explain the roles of the inositol trisphosphate receptors (IP 3 Rs) and ryanodine receptors (RyRs) in smooth muscle.
Skeletal muscle contraction is initiated by a depolarization of the surface membrane
Skeletal muscle fibers are innervated by α motor neurons, which are large neurons (cell body diameter up to 70 μm) that originate in the ventral horn of the spinal cord. As discussed in Chapter 12 , a single AP in an α motor neuron causes sufficient ACh release at a single NMJ to trigger an AP that is propagated along an entire muscle fiber.
Skeletal muscle contraction is triggered by depolarization of the muscle fiber membrane beyond a critical level, the mechanical threshold. Under normal physiological conditions an AP causes this depolarization. The relationship between V m and the amount of force, or “tension,” generated by skeletal muscle is presented in Fig. 15.1 . The mechanical responses shown in Fig. 15.1 , which are produced by depolarizations lasting several seconds, are called contractures . As V m becomes more positive than approximately –55 mV (the mechanical threshold), force increases very steeply with further depolarization. The mechanism by which depolarization of the sarcolemma causes contraction of the muscle cell is termed excitation-contraction coupling (E-C coupling) . The fact that force increases with V m indicates that the process of E-C coupling involves a voltage sensor in the sarcolemma that couples depolarization to contraction.
Skeletal muscle has a high resting Cl − permeability
In skeletal muscle, the resting V m (∼–90 mV) is much more negative than in neurons (∼–70 mV) owing to the relatively high permeability of the sarcolemma to both K + and Cl − . Because of the high resting Cl − permeability, a relatively large stimulus is normally necessary to bring the V m to mechanical threshold. In some skeletal muscle diseases, loss-of-function mutations in skeletal muscle Cl − channels drastically reduce the Cl − permeability of the sarcolemma. As a result, the skeletal muscle AP threshold can be reached more easily and the muscle becomes hyperexcitable ( Box 15.1 ).
Two inherited forms of nondystrophic myotonia congenita a involve the Cl − conductance ( g Cl ) of the skeletal muscle sarcolemma: Thomsen’s disease, with autosomal dominant inheritance; and the more severe Becker’s myotonia, with autosomal recessive inheritance. Both forms are characterized by attacks of muscle stiffness caused by a delay in muscle relaxation after stimulation. Under conditions in which normal muscle responds with a single action potential (AP), myotonic muscle cells respond to a single stimulation with repetitive APs. Thus a single α motor neuron AP evokes a twitch in normal skeletal muscle but a tetanus in myotonic muscle. A tetanus is a sustained contraction that is evoked in normal skeletal muscle only when it is stimulated by a train, or burst, of closely spaced APs ( Chapter 16 ). The rigidity and delayed relaxation of myotonic muscle are caused by the tetanic response to a single stimulus.
The hyperexcitability of myotonic muscle is caused by a mutation in the gene encoding the predominant skeletal muscle Cl − channel, CLCN1. This mutation reduces the Cl − conductance ( g Cl ) of the sarcolemma and, thus, increases membrane resistance. A stimulus will produce a larger change in V m in myotonic muscle than in normal muscle (see Ohm’s Law in Chapter 6 ). As a result, the current required to reach AP threshold is less, thereby making the muscle hyperexcitable.
A single action potential causes a brief contraction called a twitch
The temporal relationship between the skeletal muscle AP and the force generated by the muscle is illustrated in Fig. 15.2 . The skeletal muscle AP is similar to the nerve axon AP ( Chapter 7 ) in that it is generated by the activity of voltage-gated Na + and K + channels. The development of force by the myocyte begins several milliseconds after the AP. The transient contraction produced by a myocyte in response to a single AP is called a twitch . During the twitch, the contractile force rises to a peak in 30 to 50 milliseconds and then declines over the next 50 to 100 milliseconds. The duration of the twitch is approximately 100-fold longer than the duration of the AP.

How does depolarization increase intracellular [Ca 2+ ] in skeletal muscle?
In Chapter 14 we learned that an increase in [Ca 2+ ] i activates contraction in all types of muscle cells. Therefore the central question in E-C coupling is, “How does depolarization of the sarcolemma bring about an increase in [Ca 2+ ] i ?” A related, but more subtle, question is, “How does the [Ca 2+ ] i increase fast enough throughout the muscle fiber so that myofibrils deep inside the fiber can contract synchronously with those close to the surface?”
Direct mechanical interaction between sarcolemmal and sarcoplasmic reticulum membrane proteins mediates excitation-contraction coupling in skeletal muscle
In skeletal muscle, depolarization of the T-tubule membrane is required for excitation-contraction coupling
Is diffusion of a soluble factor from the surface membrane (e.g., Ca 2+ ions entering the cell through voltage-gated Ca 2+ channels) to the interior of the cell sufficiently fast to explain the rapid activation of skeletal muscle? A molecule would take about a second to diffuse to the center of a 100-μm diameter skeletal muscle cell ( Chapter 2 ). This is much too slow to account for the development of force during a twitch contraction, which begins just a few milliseconds after the AP ( Fig. 15.2 ).
How, then, does the AP in the sarcolemma rapidly activate the myofibrils in the center of the cell? The critical clue was the discovery that “hot spots” distributed over the sarcolemma (later shown to be T-tubule openings) provide a pathway for depolarization to spread from the surface into the interior of the fiber. T-tubules form an intricate network that extends throughout the skeletal muscle cell ( Fig. 15.3 ). Thus the T-tubule lumen is a narrow extension of the extracellular space into the interior of the muscle cell. The T-tubule membrane contains voltage-gated Na + and K + channels, and APs from the surface are propagated along the T-tubule membrane into the interior of the cell.

In skeletal muscle, extracellular Ca 2+ is not required for contraction
The T-tubule membrane of skeletal muscle contains receptors for the dihydropyridine derivatives that block L-type voltage-gated Ca 2+ channels ( Chapter 8 ). Voltage clamp studies in skeletal muscle demonstrate that inward Ca 2+ currents are generated by depolarization ( Fig. 15.4 ) and that these currents are blocked by dihydropyridines. Thus the dihydropyridine receptors (DHPRs) in the T-tubule membrane are L-type Ca 2+ channels. Therefore we might reasonably expect that the Ca 2+ entry through these channels raises [Ca 2+ ] i and activates contraction, but this is not the case. Skeletal muscle continues to contract normally when bathed in a solution containing no Ca 2+ ions. This finding indicates that Ca 2+ entry is not essential for skeletal muscle contraction and that all the Ca 2+ required for activating the contractile machinery is derived from intracellular sources. This is in marked contrast to the mechanism of E-C coupling in cardiac muscle, where Ca 2+ entry through voltage-gated Ca 2+ channels is required (see later). Even though Ca 2+ entry through DHPRs is not required for E-C coupling in skeletal muscle, the DHPRs do play a crucial role in this mechanism.

In skeletal muscle, the sarcoplasmic reticulum stores all the Ca 2+ needed for contraction
The SR is an extensive intracellular membrane-enclosed system of sacs and tubules that envelopes the myofibrils in skeletal muscle cells ( Fig. 14.1 ). That the SR surrounds all myofibrils has long suggested that it plays an important role in skeletal muscle contraction. The SR is a specialized ER with copious expression of three proteins: SERCA ( Chapter 11 ); the ryanodine receptor (RyR) , which is the Ca 2+ release channel in the SR membrane; and calsequestrin . Virtually all the Ca 2+ released during a contraction is rapidly and efficiently transported back into the SR by the extremely abundant SERCA in the SR membrane. The rapid removal of Ca 2+ from the cytoplasm causes relaxation. Because essentially all the Ca 2+ is recycled in this way, extracellular Ca 2+ is not required in the short term, and skeletal muscle contraction can proceed even in the absence of extracellular Ca 2+ . The capacity of the SR to store Ca 2+ is greatly enhanced by calsequestrin, the Ca 2+ -binding protein that is highly expressed in the SR lumen and acts as a Ca 2+ buffer.
The SR Ca 2+ release channels are called RyRs because they avidly bind ryanodine, a paralyzing plant alkaloid that blocks the channels. There are three RyR isoforms: RyR1 in adult skeletal muscle; RyR2 in cardiac muscle; and RyR3 in embryonic skeletal muscle, brain (in ER), and other tissues. Each Ca 2+ release channel is a tetramer of four identical RyR protein subunits. Malignant hyperthermia is a genetic disease of skeletal muscle caused by mutations in RyR1 ( Box 15.2 ).
Malignant hyperthermia (MH) is a skeletal muscle disorder characterized by hypermetabolism. MH is triggered by exposure to volatile, halogenated anesthetics, such as halothane. In children undergoing anesthesia, the incidence is 1 in 3000–15,000—about one case in a busy metropolitan hospital every other year. Although relatively rare, MH is life-threatening.
MH is characterized by an uncontrolled, sustained increase in [Ca 2+ ] i in skeletal muscle. This results in hypermetabolism: as cross-bridges continuously cycle and SERCA attempts to restore normal [Ca 2+ ] i , ATP is rapidly consumed in both processes. The maintained high [Ca 2+ ] i produces muscle rigidity. The rapid ATP consumption leads to a dramatic increase in aerobic and anaerobic metabolism, an increase in CO 2 production, and a sustained rise in body temperature. If untreated, this clinical syndrome is usually fatal. The skeletal muscle relaxant dantrolene is a lifesaving therapy. This drug, which inhibits sarcoplasmic reticulum (SR) Ca 2+ release through the RyRs, rapidly reduces the uncontrolled [Ca 2+ ] i rise in skeletal muscle and suppresses the hypermetabolism that threatens the patient with MH.
MH is genetically heterogeneous; most cases involve a point mutation in the gene encoding the skeletal muscle SR Ca 2+ release channel (RyR1). The altered channels exhibit several abnormalities that enhance Ca 2+ release from the SR. For example, MH muscle is more sensitive than normal muscle to depolarization and to caffeine, both of which open RyRs. The standard test for MH susceptibility involves measuring contracture in a muscle sample on exposure to caffeine. Compared with normal muscle, muscle from patients with MH produces larger caffeine contractures and the contractures are triggered at a lower caffeine concentration.
The triad is the structure that mediates excitation-contraction coupling in skeletal muscle
The SR and T-tubule membranes in skeletal muscle are closely apposed at specialized junctions called triads ( Fig. 15.5 ). The triad consists of a T-tubule that is flanked on either side by enlarged terminal sacs of the SR, called terminal cisterns . The RyRs are located in the membrane of the terminal cisterns. A large cytoplasmic domain of the RyR spans the narrow gap between the T-tubule and SR membranes. These domains are visible by electron microscopy and have been termed junctional feet ( Fig. 15.5 ). At the triads, the T-tubule membrane contains clusters of DHPRs that are located in precise register with the RyR subunits in the underlying SR membrane ( Fig. 15.6 ). The DHPRs are grouped into tetrads, and each of the four DHPR molecules in the T-tubule membrane is aligned with, and apposed to, one subunit of the RyR tetramer in the SR membrane. Alternate RyR tetramers are, however, unapposed by DHPRs ( Fig. 15.6 ). This precise spatial organization of RyRs and DHPRs, which does not occur in cardiac muscle, is the structural basis of the E-C coupling mechanism in skeletal muscle.


In skeletal muscle, excitation-contraction coupling is mechanical
In response to depolarization of the T-tubule membrane, the RyRs open to allow Ca 2+ to flow out of the SR, down its concentration gradient, into the cytoplasm, where it binds to TnC and activates contraction. The central question in E-C coupling is this: How does depolarization of the T-tubule membrane open the SR Ca 2+ release channel? The finding that the SR membrane is electrically isolated from the T-tubule membrane rules out the possibility that depolarization spreads directly from the T-tubule to the SR. Two classes of indirect coupling models have been proposed. First, T-tubule membrane depolarization could generate a chemical messenger that diffuses to and activates the RyRs. Alternatively, the coupling mechanism could be a mechanical link between a voltage sensor in the T-tubule membrane and the gating mechanism of the RyR in the SR membrane. The available evidence supports the mechanical coupling model in skeletal muscle.
The DHPR is homologous to the voltage-gated Na + channel. Like the Na + channel ( Chapter 5 ), the DHPR has a positively charged, membrane-spanning segment (S4 region) that acts as the voltage sensor. In the T-tubules of skeletal muscle the DHPR also acts as the voltage sensor for E-C coupling. Importantly, its function as a voltage sensor is independent of its ability to conduct Ca 2+ ions. The movement of the positive charges in the S4 segment of the DHPR in response to depolarization generates a transient capacitive current that can be measured with a voltage clamp ( Box 15.3 ). This charge movement is an essential first step in E-C coupling in skeletal muscle. The relationships among voltage-dependent activation of DHPRs, charge movement, and E-C coupling have been demonstrated in animals expressing mutated DHPRs ( Box 15.4 ). These studies suggest that the movement of the voltage sensor in the DHPR is mechanically linked to the opening of the RyR Ca 2+ release channel ( Fig. 15.7 ). The main elements of E-C coupling in skeletal muscle are illustrated in Fig. 15.8 .
In skeletal muscle, the voltage clamp can be used to measure a nonlinear component of the capacitive current that is qualitatively similar to the Na + channel gating current ( Box 7.3 ). A depolarizing voltage step generates an outward current. This current reflects the movement of charged amino acid residues in the S4 segment of the dihydropyridine receptors (DHPRs) that act as the voltage sensors for excitation-contraction coupling (E-C coupling) in skeletal muscle. Several lines of evidence indicate that this charge movement is related to E-C coupling. For example, the magnitude of the charge movement increases with the size of the depolarization and this occurs over the same range of potentials where muscle force increases ( Fig. 15.1 ). Furthermore, Ca 2+ release from the sarcoplasmic reticulum (SR) is tightly correlated with charge movement. Finally, no experimental or physiological conditions have been found that eliminate charge movement while maintaining depolarization-induced SR Ca 2+ release in skeletal muscle.
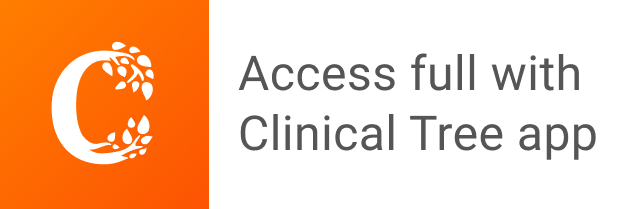