Fig. 4.1
Electrode positions and labels according to the 10–20 electrode placement system. Black circles indicate positions of the original 10–20 system, gray circles indicate additional positions introduced in the 10–10 extension (Reprint with permission from Oostenveld and Praamstra 2001)
4.2.3 EEG Rhythms
There are four basic brain waves distinguished on EEG according to their frequency ranges: delta (0.5–4 Hz), theta (4–7.5 Hz), alpha (8–13 Hz), and beta (14–30 Hz). Delta waves are primarily associated with deep sleep but may be present in the waking state. Theta waves appear as slips of consciousness toward drowsiness and have been associated with access to unconscious material, creative inspiration, and deep meditation. Alpha waves are usually found over the occipital region of the brain. Figure 4.2 shows how the wavelength and alpha frequency were calculated (Johannisson 2016). Most subjects produce some alpha waves when their eyes are closed, and these waves are dampened or eliminated by opening the eyes, hearing unfamiliar sounds, anxiety, or mental concentration or attention. Lastly, beta waves are chiefly found over the frontal and central regions and are characterized by low amplitudes.


Fig. 4.2
Alpha waves. (a) Short sequences of alpha waves . (b) An example of a sequence used in the present study. Blue tracings are from AFz-TP9 and red tracings are from AFz-TP10 (Reprint from Johannisson 2016)
In addition to the abovementioned classical waveforms, other brain waveforms have been reported (Iber et al. 2007):
- (a)
K-complexes are well-delineated negative sharp waves that are immediately followed by a positive component, usually lasting >0.5 s. K-complexes are clearly distinguishable from background EEG noise and are typically maximal in amplitude when recorded using frontal derivations.
- (b)
Vertex sharp waves are sharply contoured waves with durations <0.5 s. These waves are clearly distinguishable from background activity and are maximal in amplitude over the central region.
- (c)
Sleep spindles (also called sigma activity ) occur within the 11–15 Hz frequency range and are associated with learning, memory, and intelligence.
- (d)
Slow-wave activity describes waves of 0.5–2 Hz in frequency that have a peak-to-peak amplitude >75 μV, usually measured over frontal regions.
- (e)
Sawtooth waves are trains of sharply contoured or triangular (often serrated) 2–6 Hz waves that show maximal amplitude over central cranial regions and often, but not always, precede a burst of rapid eye movement (REM).
4.2.4 EEG Applications
Given the wealth of knowledge provided by EEG, it is no surprise that this method has paved the way for the diagnosis and treatment of many neurological disorders and abnormalities in the human body. EEG signals from humans and animals have been used for the investigation of the following clinical problems to date (Bickford 1987):
- (a)
Monitoring alertness, coma, and brain death
- (b)
Locating areas of damage following head injury, stroke, and tumor
- (c)
Testing afferent pathways (using evoked potentials)
- (d)
Monitoring cognitive engagement (alpha rhythm)
- (e)
Producing biofeedback situations
- (f)
Controlling depth of anesthesia (servo anesthesia)
- (g)
Investigating epilepsy and locating seizure origin
- (h)
Testing pro-convulsive or anticonvulsive drug effects
- (i)
Assisting the experimental cortical excision of epileptic foci
- (j)
Monitoring brain development
- (k)
Investigating sleep disorder pathophysiology
- (l)
Investigating mental disorders
- (m)
Providing a hybrid data recording system together with other imaging modalities
4.3 Applications of EEG in Acupuncture Research
EEG has long been used to understand the neurobiological basis of acupuncture and its effects (Chen and Huang 1984; Chongcheng et al. 1985). We will introduce these animal and human studies separately.
4.3.1 EEG Studies of Acupuncture in Animals
Various animal models have been employed to study the effects of acupuncture in a controlled setting. In a basic research study, He and colleagues evaluated the influence of the duration of interstitial laser acupuncture therapy on brain activity in naïve animals using EEG and HRV. Six rats underwent 10, 20, or 30 min of interstitial laser acupuncture at PC6 in a randomized order with a 30-min break between each condition. The periods of 10 min before, during, and 10 min after laser stimulation were recorded by EEG and HRV in all three conditions (10-, 20-, and 30-min laser acupuncture duration). Whereas HR was significantly altered after the 20-min red laser stimulation, neither the LF/HF ratio of HRV nor the integrated EEG showed any significant between-condition differences (He et al. 2013a). In a preclinical study, the effect of gold wire implants at specific acupoints on uncontrolled idiopathic epileptic seizures was evaluated in canines (Goiz-Marquez et al. 2009). Fifteen dogs with a positive diagnosis were enrolled in the study, and EEG recording was performed before and 15 weeks after the treatment protocol. Relative frequency power, intrahemispheric coherence available from EEG data, number of seizures, and seizure severity were compared before and after treatment using a Wilcoxon signed-rank test . No significant statistical differences were observed in relative power or intrahemispheric coherence; however, there was a significant mean reduction in seizure frequency and seizure severity after treatment. This study suggested that the effects of acupuncture on clinical presentation might not conform with characteristics on EEG.
Another study evaluated the effects of acupuncture on EEG spectral edge frequency (SEF) 95 in dogs sedated with butorphanol (Kim et al. 2006). SEF 95 values were significantly reduced during acupuncture at GV20 or at the EX-HN3 (Yintang) point and returned to the baseline values after acupuncture release. Ramsay sedation score (RSS) values also confirmed an acceptable level of sedation level during acupuncture. It was concluded that acupuncture application at GV20 or the EX-HN3 point used in combination with butorphanol sedation would be a valuable complementary method for inducing and maintaining sedation in dogs (Kim and Nam 2006).
By recording EEG signals in rats, it has found that EA stimulation of Feng-Chi acupoints is beneficial in suppressing focal epilepsy and treating epilepsy-induced sleep disruptions (Yi et al. 2015) (Fig. 4.3). The mechanism of EA in this context, however, is still unclear. One study investigated the effects of Anmian (extra) acupoint EA stimulation on sleep organization and the caudal nucleus tractus solitarius (NTS) using EEG in rats (Yi et al. 2004). One-time EA stimulation 25 min prior to the onset of the dark period enhanced REM sleep. Furthermore, the effects of EA on sleep were precluded by electrical lesion of the bilateral caudal NTS. However, EA stimulation did not alter slow-wave activity during slow-wave sleep, despite the fact that slow-wave activity was reduced after caudal NTS lesion. These results suggested that the caudal NTS might play a role in the effects of EA on sleep.


Fig. 4.3
The effect of 10-Hz EA stimulation of bilateral Feng-Chi acupoints and naloxone on epileptic activities (Reprint from Yi et al. 2015)
Panels a, b, c, and d respectively depict the EEG signals recorded from the naïve rats, the pilocarpine group, the PFS (pyrogen-free saline) + EA + pilocarpine group, and the naloxone + EA + pilocarpine group, beginning from the dark onset of the dark period. Pilocarpine was administered at time 0 in the left panels of b, c, and d. The blue boxes represent the epileptiform EEGs. Red lines indicate the extracted time points for the expanded time-scale figures in the right panels. Green arrowheads are the artifacts. The larger amplitudes, with EEG signals less than 2 mV that appeared in panels a, were delta waves, which represent the state of slow-wave sleep.
Intravenous (i.v.) laser blood irradiation using a one-way catheter (Gamaleia et al. 1988; Korochkin et al. 1988) and percutaneous interstitial (i.st.) laser therapy using a sterile catheter permit the penetration of laser light into deeper tissues for the treatment of herniated disks or spinal stenosis (Weber 2011). Intravenous (i.v.) laser blood irradiation, interstitial (i.st.) laser acupuncture, and EA were compared using EEG and HRV and were investigated in ten anesthetized male Sprague-Dawley rats. HR was significantly decreased during i.st. laser acupuncture stimulation of Neiguan, while total HRV was nonsignificantly increased during i.v. and i.st. laser stimulation. The LF/HF ratio only showed significant changes during i.v. laser blood irradiation. Integrated cortical EEG showed insignificant decreases during EA and i.v. laser blood irradiation. Further studies concerning the dose-dependent nature of these effects are in progress (He et al. 2013b ).
4.3.2 EEG Studies of Acupuncture in Humans
Studies of healthy volunteers. A number of acupoints had been studied by using EEG to evaluate the specific changes in spontaneous electrical activity in the brain. For example, one study investigated the effects of acupressure at the Extra 1 point on EEG spectral entropy values and heart rate variability (HRV). Compared with sham control, acupressure significantly reduced EEG spectral entropy and decreased the low-frequency/high-frequency (LF/HF) ratio of HRV (Arai et al. 2011). Physiological responses to electroacupuncture (EA) stimulation of acupoints PC5 and PC6 have also been evaluated using EEG and HRV; EA stimulation of these points increased the number of low-frequency waves in all lobes and additionally increased the mean R-R interval (Kim et al. 2009). Manual acupuncture at LI4 was shown to significantly increase the alpha-1 frequency and shifted the ratio of alpha-1/theta to favor alpha-1 at all electrodes (Juel et al. 2016). HRV parameters in this study showed a significant increase in the LF/HF ratio during the first minute of LI4 stimulation and followed by a decrease thereafter. These studies suggest that various acupoint locations and stimulation methods might lead to alterations in EEG and HRV signal.
Distinct analysis methods have been employed in the study of acupuncture. A wavelet-limited penetrable visibility graph approach was used to analyze EEG data from 15 healthy subjects undergoing acupuncture at acupoint ST36 . The study found that acupuncture influenced the complexity of EEG sub-bands in different ways and led to higher efficiency and stronger small-world properties of functional brain networks compared with the pre-acupuncture control state (Pei et al. 2014). Another study used an order recurrence quantification analysis combined with discrete wavelet transforms to analyze the dynamic characteristics of different EEG rhythms during ST36 stimulation. Stimulation decreased the complexity of the delta rhythm, increased that of the alpha rhythm, and had no obvious effects on the beta, theta, and gamma rhythms relative to the pre-acupuncture state (Yi et al. 2013).
Magnetic acupuncture is a painless stimulation method and has been recently reported as an effective modality in clinical practice (Colbert et al. 2008). The effects of magnetic stimulation at acupoint HT7 were evaluated by examining event-related potentials (ERPs) on EEG. Colbert and colleagues identified an obvious P150 component in response to magnetic acupuncture using the dipole model. Acupuncture at HT7 was found to evoke stronger activity in the somatosensory cortex than sham stimulation and sham acupoint stimulation (Geng and Zhang 2012). Magnetic LI4 stimulation was also investigated using EEG; a peak potential was recorded at frontal midline (FCZ) electrode sites at about 140–170 ms (likely to be P150) after acupoint stimulation but not non-acupoint stimulation (Yu et al. 2009).
Another study explored the differential effects of acupressure, acupuncture, and laser acupuncture on EEG bispectral index, spectral edge frequency, and verbal sedation score using the acupoint EX-HN3 and a sham point. Bispectral index and spectral edge frequency values both significantly decreased during acupressure stimulation at EX-HN3, and all three interventions significantly reduced verbal sedation score (Litscher 2004).
The effects of laser acupuncture, manual acupuncture, and light stimulation on cerebral blood flow were investigated in 15 healthy volunteers using noninvasive transcranial Doppler sonography and the bispectral index of EEG (Litscher et al. 2000). Light stimulation significantly increased blood flow velocity in the posterior cerebral artery, while a similar but less pronounced effect was seen after manual and laser acupuncture at vision-related acupoints. Furthermore, significant increases in the amplitudes of 40-Hz cerebral oscillations were noted during both laser and manual acupuncture.
The effects of acupuncture at PC6 have also been assessed. Chang et al. reported an increase in the amplitude and power of the alpha band during manual PC6 acupuncture compared to baseline data (Chang et al. 2009). Meanwhile, frequency peaks in the alpha bands of 12 channels were all synchronized with a much smaller standard deviation compared with the baseline data, and these phenomena persisted for at least 10 min after the cessation of stimulation. Kim and his colleagues found the similar results that the power of the frequency bands including alpha-wave, beta-wave, theta-wave, and delta-wave all increased considerably after acupuncture stimulation at PC6 (Kim et al. 2008).
The effects of high- (100 Hz) and low-frequency (2 Hz) transcutaneous electrical nerve stimulation (TENS) at LI4 were compared for the delta, theta, alpha-1, alpha-2, beta, and gamma bands using 124-channel EEG (Chen et al. 2006). The absolute EEG powers (muv2) at focal maxima across three stages (baseline, stimulation, and post-stimulation) were examined by using two-way (condition, stage) repeated measures ANOVA. Theta power activity was significantly decreased during high-frequency but not low-frequency acupoint stimulation relative to control stimulation. Moreover, decreased theta power was prominent at the FCZ and contralateral right hemisphere frontal (FCC2h) areas during high-frequency simulation.
It was previously reported that subject pain perception combined with the corresponding EEG waveforms could be used to describe the body’s systemic neural response to a given stimulation (Kakigi et al. 2005). One study evaluated the statistical relationship between brain activity and the experience of pain induced by GB34 stimulation with intensive light impulses (wavelength, 500–1200 nm) (Lee et al. 2011). EEG data were recorded from sites F(p1) and F(p2). According to the results, the area under the curve of brain waves induced by pain was useful as a proportional indicator of pain perception.
Another study explored the relationship between electrical activity changes measured with EEG and patient experiences during acupuncture using items of the acupuncture sensation questionnaire (Yin et al. 2010). The results indicated that only the group with higher ratings of needle sensation showed significant changes in alpha band EEG powers over the periods before, during, and after needle insertion. Accordingly, acupuncture effects on EEG activity may closely relate to needle sensation in some patients.
Studies of acupuncture on healthy subjects have mainly explored the specificity of acupuncture according to the principles of Traditional Chinese Medicine by examining acupoint specificity, acupuncture sensation, and different responses induced by distinct stimulation methods. However, the findings are difficult to synthesize and even contradictory in many cases due to inter-study differences in experimental design, small sample sizes, and the details of acupoint stimulation.
Studies in patients with indications for acupuncture. Intraoperative transcutaneous acupoint electrical stimulation (TAES) has been reported to improve the sedative effect of propofol, a widely used sedative anesthetic agent (Nayak et al. 2008; Ding et al. 2013). Further, TAES has also been reported to reduce post-operative opioid intake and decrease the incidence side effects related to anesthesia while improving the quality of recovery from anesthesia (Wang et al. 1997, 2014). The mechanism of TAES and its beneficial interactions with anesthetics was investigated in a previous EEG oscillation analysis (Liu et al. 2016). EEG was continuously measured during both light and deep propofol sedation (target-controlled infusion set at 1.0 and 3.0 μg/mL, respectively) in ten patients undergoing surgery. Propofol infusion was maintained for 6 min, and each 6-min period was divided into three 2-min phases. TAES was initiated between 3–4 min after initiation of the propofol infusion. EEG power spectrum changes in different frequency bands (delta, theta, alpha, beta, and gamma) and the coherence of different EEG channels were analyzed. The result showed that after TAES application, EEG power was increased in the alpha and beta bands during light sedation, but was reduced in the delta and beta bands during deep sedation (Fig. 4.4). In addition, the EEG oscillation analysis showed that TEAS increased synchronization at low frequencies and decreased synchronization at high frequencies during light or deep propofol sedation (Fig. 4.5). Accordingly, while this study suggested that TAES might improve the effects of propofol during light sedation, it is hard to conclude that TAES is beneficial in combination with propofol anesthesia without further corroboration of these findings.



Fig. 4.4
Topoplot of EEG powers in different frequency bands during different phases during (a) light propofol sedation and (b) deep propofol sedation (Reprint from Liu et al. 2016). Transcutaneous acupoint electrical stimulation was applied during phases 2 and 5

Fig. 4.5
The effects of transcutaneous acupoint electrical stimulation (TAES) on synchronization among EEG channels at different frequency bands during propofol administration at 1 μg/mL (a) and 3 μg/mL (b) (Reprint from Liu et al. 2016). Red nodes indicate cortical electrodes. Lines between nodes indicate significant changes in synchronization between the two channels before and after TAES. The color of the line represents the strength of synchronization (red indicates increased synchronization and blue indicates decreased synchronization)
Silva and colleagues analyzed the efficacy of TENS for post-mastectomy pain and its ability to produce eletrocortical changes in somatosensory areas (Silva et al. 2014). EEG was recorded in absolute power in the alpha band (8–14 Hz), and pain assessments were conducted before and after TENS intervention. TENS produced decreases in both slow (8–10 Hz) and fast alpha (10–12 Hz) wavebands and led to 88.4% reduction of pain scores. Accordingly, it was concluded that TENS supported the modulation of electrical stimulation in the parietal region and reduced clinical post-mastectomy pain.
In conclusion, EEG is often used to diagnose disorders that influence brain activity, such as epilepsy (Chu et al. 1991; Chu 1992), dementia (Gao et al. 2001), and attention-deficit hyperactivity disorder (Arnold 2001). Yet, the number of acupuncture clinical trials using EEG is relatively small. Future studies should employ EEG in the assessment of acupuncture in patients with acupuncture indications in order to better elucidate possible mechanisms of action.
In summary, EEG is a convenient and safe method that is widely used for preclinical and clinical research. Notably, EEG can provide precise temporal dynamic information about the effects and mechanisms of action of acupuncture. A majority of studies to date has focused on evaluating the characteristics of acupuncture in humans and various animal models. However, the conclusions of these studies have been inconsistent; the differences of stimulation acupoints, stimulation types, and experimental design might contribute to the inconformity.
4.4 Principles of MEG
Neural activity in the brain gives rise to electrical currents that spread in the surrounding volume conductor and produces volume currents that partially reach the surface of the scalp. Volume currents are passive in nature and behave, on a macroscopic scale, according to Ohm’s law. Volume currents are generated in response to primary currents, which flow inside of or in the vicinity of neurons (Lutkenhoner 2003). Accordingly, both primary currents and volume currents contribute to magnetic fields measured by MEG. That is, when information is being processed, sufficient current flow can produce a weak magnetic field that can be measured noninvasively using a magnetometer placed on the outside of the skull. However, magnetic fields only reflect activity in the uppermost layer of the brain, the cerebral cortex, which is a 2–4-mm thick sheet of gray matter tissue. Although MEG is a relatively new method of recording, it has already facilitated novel research on the functioning of the human brain and is expected to become increasingly important in the near future as clinical applications for MEG emerge.
Regions of the brain that are activated in response to a given stimulus or during the resting state can be localized based on the observation of magnetic fields using MEG. In this case, a typical MEG signal can be evoked by a tone burst.
4.4.1 Basic Principles of MEG
Sensory stimuli initially activate small pertinent portions of the cortex. This process is associated with primary current generation related to the movement of ions across neuronal membranes according to their chemical concentration gradients. In addition, passive ohmic currents (volume current) are produced in the surrounding medium. This volume current completes the loop of ionic flow so that there is no buildup of charge. Together, primary current and volume current lead to the generation of a magnetic field. If the primary source and surrounding conductivity distribution are known, the resulting magnetic field can be calculated from Maxwell’s equations as follows:





(4.1)

(4.2)

(4.3)

(4.4)
where
represents the electric field,
represents the magnetic field, ρ represents the volume density of free charges, ε 0 represents the mediated rate, and μ 0 represents magnetic permeability.


In certain finite conductor geometries, the volume current causes the generation of an equivalent and opposite field to the primary current. The net external field is then zero. Therefore, MEG can only measure activity from fissures of the cortex. Because all primary sensory areas of the brain (auditory, somatosensory, and visual) are located within fissures, MEG can be used to study functional brain responses to a variety of stimuli.
4.4.1.1 The Current Dipole Source Model
Current dipole is a popular source model in MEG research that is used to approximate the flow of electrical current in small areas. The typical strength of a dipole caused by the synchronous activity of what is likely to be tens of thousands of neurons is 10 nA m. For a single current dipole source, the map of the radial magnetic field B has one maximum and one minimum. The dipole is half of the field extremum at a right angle to the line joining the maximum and minimum. The magnitude, direction, and position of the source can be deduced unambiguously from this map, provided that the dipolar assumption is valid, i.e., the source currents are limited to a small region of the brain. This model provides a basis for the consideration of more complex source constellations. In principle, any conceivable distribution of currents in the brain can be represented in terms of current dipoles. In addition, no more than six parameters are required to define this model: the three coordinates of the dipole location and the three components of the dipole moment. The latter is a vector having the same orientation as the mean current in the source. The length of this vector corresponds to the strength of the current multiplied by a measure of length, which characterizes the mean distance over which the current flows.
The magnetic field arising from a current dipole has a characteristic pattern. To illustrate this point, the contours of the human head are shown in Fig. 4.6. The bulky short arrow represents a current dipole located 6 cm below the measurement surface. This dipole is aligned with the x-axis, which runs from the center of the head to the nasion. The normal component of the magnetic field arising from this dipole is visualized as a topographical map; magnetic flux directed out of the head (positive polarity) is shown in the left hemisphere, whereas magnetic flux directed into the head is shown in the right hemisphere. In between these two hemispheres is the median plane, where the flux is zero. The two field extrema are located in a plane running orthogonal to the median plane through the y-axis.


Fig. 4.6
Contours of a human head with a topographical map visualizing the normal component of a magnetic field arising from a current dipole (Lutkenhoner 2003). The dipole (thick gray arrow) is located 6 cm below the measurement surface (sphere with a radius of 12 cm). The two field extrema are represented by filled circles. The symbols indicate the polarity of the magnetic field: + for magnetic flux out of the head and − for magnetic flux into the head (Reprint with permission from Wikswo and Roth 1988)
4.4.2 Measurement of MEG Signal
Compared to potentials recorded at the scalp, which depend on the conductivities of the brain, skull, and scalp, magnetic field is able to pass through intervening tissues unchanged; this means that the head is essentially transparent to magnetic fields. However, it is known that the magnetic field component perpendicular to the scalp (normal component) is not very sensitive to volume currents. Thus, detailed information about the volume conductor is often dispensable for data analysis. This is one reason why MEG measurements favor the normal component of the magnetic field.
MEG signal is typically measured at a distance of about 2 cm from the scalp using a sensitive superconducting quantum interference device (SQUID) detector (Kim et al. 2014). Because magnetic signals from the brain are extremely weak compared with ambient magnetic-field variations, the elimination of outside disturbances is of utmost importance. For example, the electrical activity of the heart generates a magnetic field that is 2–3 orders of magnitude larger than signals originating from the brain. To this end, the sensitivity of SQUID detectors to external magnetic noise is greatly reduced by proper design of the Aux transformer, a device that facilitates magnetic signal detection by the SQUID. In addition, MEG measurements are usually performed in a magnetically shielded room.
4.4.3 MEG Data Processing
The neural processing of incoming information can be studied by recording responses to sensory stimuli. In many experiments, spontaneous brain activity and incoherent background events can be sources of noise. Thus, signals resulting from many successive stimuli must be averaged to isolate evoked responses. For localization of the active brain area, measurements are made over several sites, typically 20–60 points across the scalp separated by about 3 cm. At each location, the stimulus must be repeated 20–500 times in order to produce an adequate signal-to-noise ratio. This is a potentially tedious and time-consuming procedure that also endangers the reliability of the data: the subject cannot be expected to remain in the same state of vigilance throughout long measurement sessions.
Indeed, the primary drawback of MEG in early studies was the amount of time required to gather sufficient data for topographical field map generation. To minimize the amount of time required, several MEG research groups and companies are already constructing and using multi-SQUID magnetometers . For example, a 24-channel neurogradiometer employing planar gradiometric flux transformers was recently developed to provide accelerated data acquisition.
Another factor seriously influencing the quality of MEG signal is the distance between the source and the center of the head. It is useful to consider the idealized case of a volume conductor with spherical symmetry; for such a volume conductor, the amplitude of the MEG signal does not only decrease with the squared distance between source and measurement site, but is, in addition, proportional to the distance between the source and the center of the sphere (Lutkenhoner 1996). Thus, a source in the center is magnetically silent. In practice, a deep source is not completely silent on MEG, but can be expected to produce a magnetic field that is about one order of magnitude smaller than that of a comparable source located close to the surface of the brain (Menninghaus and Lutkenhoner 1995). There is opportunity for the improvement of this aspect of MEG, but additional studies are required to facilitate this advancement.
4.4.3.1 The Inverse Problem
Given a complete knowledge of currents and a sufficiently realistic volume conductor model, it is relatively easy to calculate the associated magnetic field. This matter is often called the forward problem. However, in practice, the problem is reversed: the magnetic field is given, and the task is to estimate the underlying currents in the brain. Unfortunately, this inverse problem has no unique solution; in principle, each magnetic field has an infinite number of possible interpretations.
When put into use, a single current dipole is rarely a perfect model, which means that at least one additional dipole is required to explain the data. Yet, it is often difficult to ascertain how many additional dipoles are required, given that successively increasing the number of dipoles rarely results in a sharp transition from an insufficient match to an almost perfect match between model and data. In addition, the fact that the number of model parameters often exceeds the number of independent data values is also a problem. To mitigate this situation, several methods have been developed, such as the parametric method, which assumes that a source consists of a few current dipoles, and the imaging method, which is based on the idea of a continuous distribution of main currents throughout the whole brain (Baillet et al. 2001). Each method has its specific advantages and disadvantages (Kaufman et al. 1990).
4.4.3.2 MEG Resolution Issues
The time resolution of MEG is better than 1 ms, and the spatial or imaging resolution is (under favorable circumstances) 2–3 mm for sources in the human brain. Spatial resolution is defined as the spatial limit (distance) between two sources that permits the independent resolution of each source. However, in practice, it is not impossible to characterize the spatial resolution of a given method using a single number, since numerous factors need be taken into consideration (Supek and Aine 1993). It has been suggested that, as a rule of thumb, two sources can only be resolved and separated if the intersource distance is at least of the same order of magnitude as the distance between the sources and measurement locations (Tan et al. 1990). This rule is consistent with the finding that it is difficult to calculate the spatial extent of a source from MEG measurements whenever the source-to-coil distance is much larger than the dimensions of the source (Wikswo and Roth 1988).
Spatial or imaging resolution is not to be confused with localizing resolution, which refers to the accuracy with which a particular source can be localized by a given method (Dale and Halgren 2001; Florin and Baillet 2015). Although the localizing resolution has no principle limitation, limitations in practice are imposed by noise in the data as well as model inaccuracies. The localizing resolution of MEG under favorable circumstances makes it useful for identifying functional landmarks in the brain, which can be of value in stereotactic and functional neurosurgery (Orrison 1999).
Imaging methods (discussed in the previous section) tend to identify sources as relatively widespread, even when the sources themselves are small or relatively specific. This behavior can be characterized by the point spread function, which describes how activity at a distinct point in the brain affects estimated activity in other locations (Liu et al. 2002). Another measure characterizing the spatial resolution of a method is the resolution field (Lutkenhoner 2001; Liu et al. 2002); the resolution field specifies how activity estimated for a certain point in the brain is affected by activities at other locations. The resolution field is not only defined for imaging methods but also for parametric methods.
4.4.4 Characterization of MEG Data in Different Frequency Bands
MEG data is collected in several different frequency bands; that is, analyses are usually performed for the most commonly used frequency bands in order to facilitate comparisons with other studies. These bands include the delta (0.5–4 Hz), theta (4–8 Hz), alpha (8–12 Hz), beta (13–30 Hz), and gamma (30–45 Hz) frequency bands (Siebenhuhner et al. 2013; Bajo et al. 2015; Kotini and Anninos 2016). Data in each frequency band provides information about different characteristics of human brain functions.
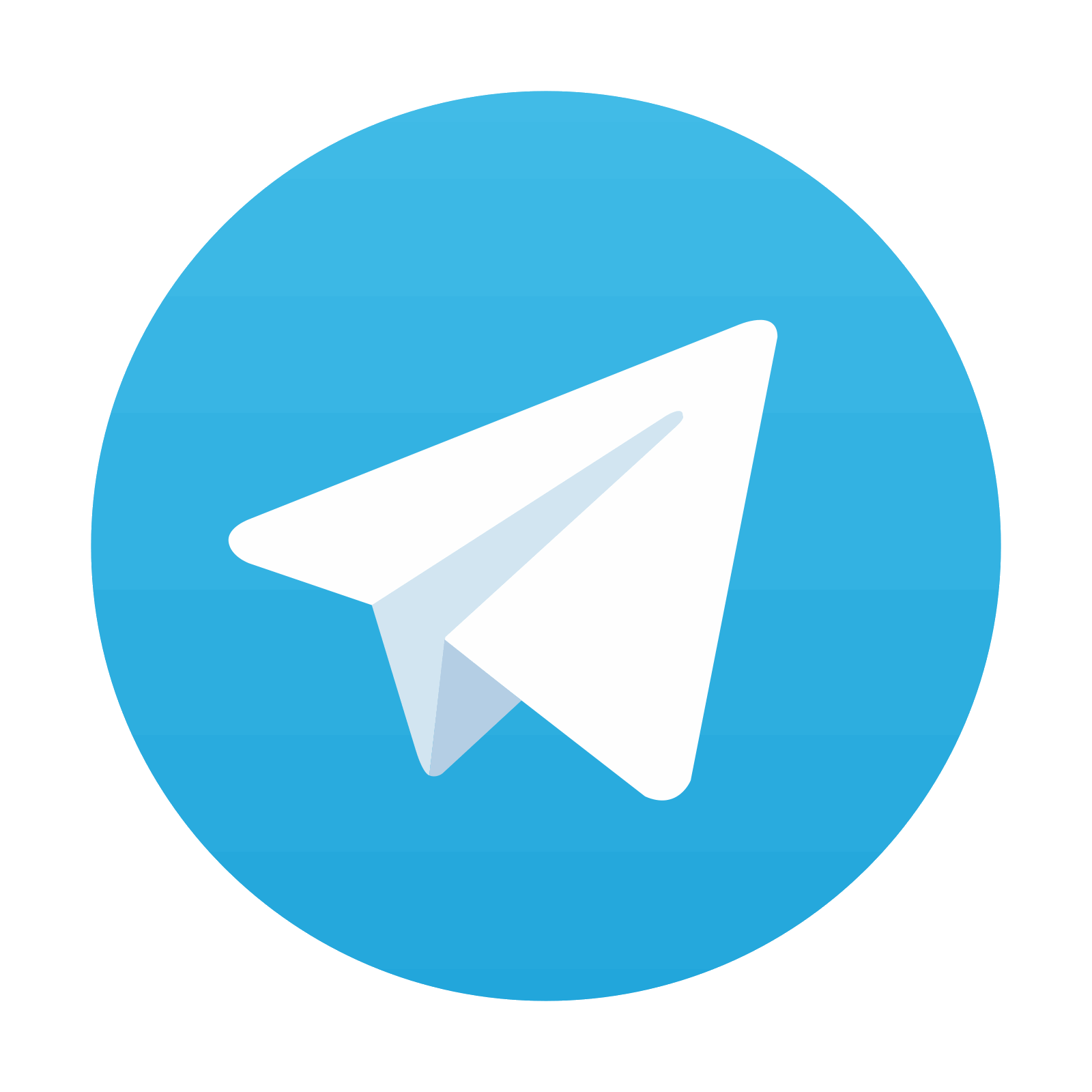
Stay updated, free articles. Join our Telegram channel
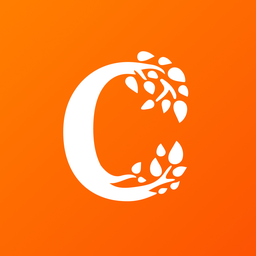
Full access? Get Clinical Tree
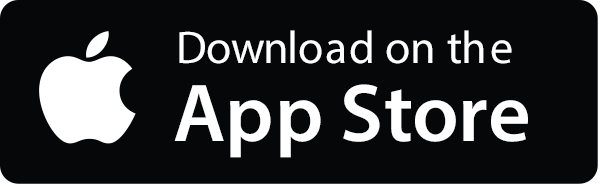
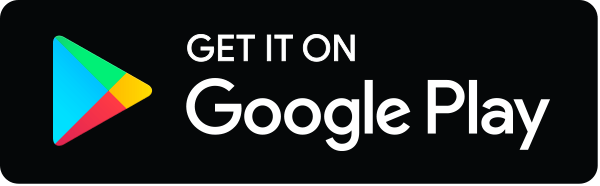