, Hyun Ho Jung1 and Jin Woo Chang1
(1)
Department of Neurosurgery, Brain Research Institute, Yonsei University College of Medicine, Seoul, Korea
Obsessive-compulsive disorder (OCD) is a disabling neuropsychiatric disorder characterized by recurrent and intrusive thoughts, desires, and/or images (obsessions ) that lead to repetitive behavior aimed at reducing the associated anxiety (compulsions ). Typical symptoms of OCD include fear of being contaminated by the environment, which patients try to ease by compulsively washing their hands until they become sore and chapped. Despite this effort, obsessive thoughts and the resulting behavior persist. Epidemiologic studies have revealed that about 1–2 % of the general population meet the diagnostic criteria for OCD at some time in their lives, with a lifetime prevalence of 2–3 % [4, 24, 27]. Despite the best available treatments including cognitive-behavioral therapy and medications, 10–20 % of patients with OCD remain refractory to conservative treatments [54].
Various neurosurgical approaches such as ablative procedures, deep brain stimulation (DBS), and gamma knife radiosurgery (GKRS) have been used for treating refractory OCD patients. Recently, transcranial magnetic resonance-guided focused ultrasound (MRgFUS) has been introduced as a novel thermal ablation method that is performed without opening the cranium [12, 26, 32, 36]. The appropriate targets, surgical procedures, technical considerations, advantages, potential risks, and clinical results of MRgFUS for OCD, will be discussed here.
12.1 Contemporary Neural Circuits in Obsessive-Compulsive Disorder
Although contemporary understanding of the neural circuits involved in psychiatric disorders is advancing rapidly, the exact neural circuits related to OCD are yet to be fully elucidated. Many earlier studies have revealed increased activity within the orbitofrontal cortex, anterior cingulate gyrus, and caudate nucleus [2, 45], whereas decreased activity has been observed in the dorsolateral prefrontal cortex [52]. A correlation has also been reported between decreased metabolism in the caudate nucleus and improvements in OCD symptoms after medical treatment [23]. Dysregulation of basal ganglia circuits has been demonstrated in the motor and OCD symptoms of Tourette syndrome . These circuits and some of the clinical features of OCD are also related to those of Parkinson’s disease [30, 38]. These findings support the hypothesis that cortico-striato-thalamo-cortical (CSTC) loop functions are strongly implicated in the pathogenesis of OCD [3, 30]. Decreased frontal-striatal control of limbic structures such as the amygdala results in an inadequate fear response in patients with OCD and may also be responsible for the fear of contamination [52].
With this understanding of neural circuitry, three interconnected neural circuits have been proposed in the pathogenesis of OCD: the circuit of Papez, the basolateral circuit, and the CSTC loop [3]. The anterior nucleus of the thalamus projects to the cingulate gyrus through the anterior limb of the internal capsule (ALIC) in the circuit of Papez [25]. The dorsomedial nucleus of the thalamus projects to the orbitofrontal cortex via the ALIC in the basolateral circuit [3]. The thalamus also projects to the cerebral cortex via the ALIC in the CSTC loop, and these anatomical locations, together with the anterior cingulate gyrus and nucleus accumbens , are the most frequent targets in surgical treatments for OCD. Thus, knowledge of these interconnected neural circuits is critical for understanding the rationale behind the choice of certain targets for surgical intervention.
12.2 Surgical Strategies for Obsessive-Compulsive Disorder
Surgical strategies can be divided into two categories: ablation and neuromodulation . Various anatomical targets have been proposed for either approach. However, there are still controversies in the application of surgical procedures to anatomical targets in patients with OCD.
Ablative procedures offer benefits to about 45–65 % of patients with intractable OCD [21]. Anatomical targets include the anterior cingulate gyrus and anterior limb of the internal capsule. Considering the major role of the anterior cingulate gyrus in the neural circuits described above, neurosurgeons can target this structure for ablative therapy in patients with OCD. We have previously reported that bilateral anterior cingulotomy resulted in a mean improvement of 36 % in the Yale-Brown obsessive-compulsive scale (Y-BOCS). Among 14 cingulotomy patients, 6 met the criteria for responders, with a 35 % or higher improvement rating on Y-BOCS 12 months postoperatively. Most importantly, there was no significant cognitive dysfunction after cingulotomy [28]. Similar results have been reported after anterior capsulotomy , with a mean improvement in Y-BOCS of 33 % and no adverse cognitive effects [40, 42]. The invasive nature of ablative procedures means they can inevitably be associated with surgical complications such as intracerebral hemorrhage, epilepsy, and hydrocephalus. In addition, the operator cannot always estimate the exact size and location of the lesions until they are visualized in postoperative images. The development of GKRS has provided a non-invasive and accurate method for locating lesions without the need for invasive surgery. However, the use of a high dose of radiation is associated with an unpredictable risk of adverse effects [48, 49].
Several deep brain structures including the ALIC, nucleus accumbens (NAc), caudate nucleus , subthalamic nucleus (STN), and dorsomedial thalamus have been proposed as DBS targets. Nuttin et al. [39] reported a double-blinded study that achieved an improvement in Y-BOCS score of at least 35 % in 50 % of patients after bilateral anterior capsular DBS. The mean Y-BOCS score was 19.8 ± 8.0 during stimulation compared with 32.3 ± 3.9 with the stimulator turned off, and this stimulation-induced effect was maintained for at least 21 months after anterior capsular DBS. Along with the improvements seen after DBS of the anterior capsule, the ventral caudate nucleus [1] and NAc [50] have also been proposed as effective DBS targets in treatment-refractory patients with OCD and have yielded improvements in OCD symptoms. Unlike ablative procedures, DBS is reversible and adjustable based on symptoms or disease progression. However, DBS requires permanent implantation of at least one multi-contact electrode, lead extensions, and an implantable pulse generator (IPG). This means that the patient must be followed closely for device management, and undergo replacement of the IPG every 3–5 years. Furthermore, DBS devices are sensitive to high-energy electrical fields, which can switch them off or even cause a reset of the device. Despite the relatively effective outcomes described above, these limitations have urged investigators to find less-invasive and more precise methods.
12.3 Transcranial Magnetic Resonance Guided Focused Ultrasound
12.3.1 Brief History
The earliest applications of ultrasound in medicine were therapeutic. Initial reports on the biological effects of ultrasound appeared early in 1928 when Harvey and Loomis reported that high-intensity high-frequency ultrasound could bring about changes in living biological tissues [43, 51]. Although a few studies reported successful treatment outcomes using ultrasound [13, 47], therapeutic applications were hampered until the late 1990s, especially due to safety issues. With the development of improved ultrasound techniques and greater understanding of the effects of ultrasound on cells and tissues, such as the damage mechanisms, thresholds, and propagation properties through tissues, the therapeutic application of ultrasound has been realized [43].
Transcranial ultrasound has been used in pediatric neurosurgery to detect hydrocephalus and midline shifts in the brain. In adults, blood velocity in the carotid arteries may be monitored through the intact skull using the Doppler effect . Lynn et al. [34, 35] reported the earliest investigation of focused ultrasound for non-invasive ablation in 1942. Since the 1950s, the use of focused ultrasound to produce focal thermal lesions deep within the brain has been demonstrated in several studies [16–18]. Fry et al. [14] demonstrated that low-frequency (around 0.5 MHz) ultrasound could be focused through the skull. Later, this group also demonstrated the acoustic parameters and histological features of focal brain lesions produced in 10 craniectomized cats using intense focused ultrasonic beams [15]. Because the histological appearance of these lesions compared with previous thermal lesions was similar without the intervening skull, they predicted the application of transcranial ultrasound in the clinical field.
Until the 1990s, the major obstacle to the use of transcranial focused ultrasound was the skull itself. Its deflecting effects and variable thickness affect wave propagation to such an extent that ultrasound could not produce a focal lesion. The generation of high temperatures within the skull was also a major concern due to energy absorption, which could damage the scalp, bone, and adjacent brain parenchyma. For this reason, previous focused ultrasound treatment of the brain required removal of the skull for energy delivery, resulting in an invasive procedure with additional risks and costs [14, 15, 17, 22]. As a result, many researchers agreed that therapeutic ultrasound energy could not be delivered through an intact skull. However, the development of phased array transducers compatible with magnetic resonance, which is the most progressive technological advancement, has rekindled interest in transcranial focused ultrasound. Combined with the utilization of temperature-dependent proton resonance frequency shift, which allows MR thermometry, these advances have yielded MRgFUS technology, which enables non-invasive, image-guided, and temperature-monitored MRgFUS interventions [29].
12.3.2 Biological Effects of Ultrasound
It was originally believed that diagnostic ultrasound would never produce biological damage. However, in the early 1990s, Child et al. [6] reported that diagnostic ultrasound could produce significant damage in mice. Two main biological effects are demonstrated when high intensity acoustic waves propagate through tissues: thermal, and non-thermal or mechanical effects [41, 43].
12.3.2.1 Thermal Effects
The principle of focused ultrasound is that a beam of ultrasound is brought to a focus on the target. As ultrasound waves propagate into attenuating materials such as tissue, the wave amplitude decreases with distance. This attenuation is caused by wave absorption and scattering. Whereas absorption is thought of as a mechanism that converts some portion of the ultrasonic wave into heat, scattering can be thought of as changes to the direction of the wave. Wherever absorption takes place in tissues, the temperature will increase as long as the rate of heat production is greater than the rate of heat removal [41]. The thermal mechanism is relatively well understood because the increase in temperature produced by ultrasound can be calculated using the widely used bio-heat transfer equation [43]. With this equation, it is possible to estimate the thermal dose and evaluate whether the dose is high enough to destroy the tissue. Injurious effects in vitro generally occur at temperatures of 39–43 °C after a sufficient time period; at higher temperatures (≥44 °C) coagulation of proteins can occur [41]. Dickson and Calderwood [11] reported that exposures of long duration (5–100 h) were required for thermally induced cell death at 40 °C and no irreversible adverse effects were detected at temperatures below 40 °C.
The temperature of the focused volume may rise rapidly by more than 20 °C. Although the volume of destroyed tissue is small (typically 0.5 mL) for a single ultrasound beam, a more clinically relevant volume can be obtained from contiguous arrays of focused ultrasound lesions throughout the volume of interest [51].
12.3.2.2 Non-thermal or Mechanical Effects
Of the non-thermal effects, acoustically generated cavitation has received the most attention, and results principally from microbubbles in ultrasound contrast agents. Radiation forces can also be produced by the non-thermal effects of ultrasound.
Acoustic cavitation refers to ultrasonically induced activity occurring in a liquid or liquid-like material containing microbubbles that are either formed spontaneously or are present naturally. Under ultrasonic stimulation, these microbubbles oscillate and can collapse at sufficiently high ultrasonic pressure levels. This phenomenon is termed inertial cavitation because the bubble motion is dominated by the inertia of the liquid [41]. In general, inertial cavitation results when microbubbles expand during the acoustic cycle and then collapse rapidly due to oscillations and the rapid growth of the cavity [43]. This cavitation can generate a temperature increase, mechanical stress, and reactive free radicals [41]. Microbubble oscillation itself also produces mechanical stress due to the viscosity of the surrounding fluid, which opposes the oscillation, creating what are known as radiation forces [43]. The occurrence of cavitation and radiation forces depends precisely on the type of tissue being exposed, making it difficult to obtain a consistent response.
12.3.3 The MRgFUS System
For non-invasive, non-ionized thermal ablation with real time imaging and thermal feedback, MRgFUS is an attractive modality. This technique addresses the limitations described above by combining a large phased array, active water-cooling, an acoustic aberration correction algorithm, and computed tomography (CT) data for skull-thickness registration.
The large phased array transducer used in MRgFUS is composed of numerous transducer elements. It has been proven that large hemispherical phased arrays can deliver adequate energy through human skulls to ablate brain tissue in vivo without an excessive elevation of temperature on the skull surface [7, 8]. Current MRgFUS systems employ 1,024 hemispherical phased array transducers operating at a frequency of 650 kHz. The interface between the subject’s head and the transducer is also filled with water to provide the acoustic path. The MRgFUS system includes a chiller (refrigeration unit) that keeps the water chilled to a constant temperature so that the skull-bone temperature remains within safe limits.
Acoustic aberration is created mostly by variations in the bony structure of the skull. The degree of compensation necessary for each transducer element is based on predicting the aberration along the acoustic path from each element to the target and calculating the relative phase and amplitude correction necessary for that element. The result of this compensation is that the acoustic energy contribution from each element will arrive at the focal point in phase. The phase/amplitude correction algorithm, based on ray acoustic methods, relies on an input that provides the bone density profile along a ray cast between each acoustic element and the target point. This information is extracted from a three-dimensional CT image of the skull.
The MRgFUS system combines a focused ultrasound delivery system with a conventional diagnostic 1.5 T or 3 T MRI scanner. This system provides a real-time therapy planning algorithm, thermal dosimetry, and closed-loop therapy control. The treatment process for MRgFUS is not very different conceptually from the system that is currently in clinical use for other soft tissue applications. The treatment begins with a series of standard diagnostic MR images to identify the location and shape of the area to be treated. The workstation uses the physician’s designation of the target volume to plan the best way to cover the target volume with small spots called “sonications”. These treatment spots are cylindrical in shape. Their size depends on the sonication power and duration. During the treatment, a specific MR scan, which can be processed to identify changes in tissue temperature, provides a thermal map of the treatment volume to confirm the therapeutic effect [53]. The thermal map is used to monitor the treatment in progress, and confirm that the ablation is proceeding according to plan, thus closing the therapy loop.
Before delivering a therapeutic dose of acoustic energy to the target site, confirmation of the alignment of the thermal spot within the target site is necessary. Therefore, several sub-threshold sonications (low power and short duration, usually 10–20 s) should be performed for which the peak tissue temperature (39–42 °C) is below the threshold for ablation but can still be visualized on MR thermography images. After this targeting confirmation procedure, sequential sonications of incremental acoustic energy levels can be applied to the site to induce tissue ablation, as indicated by peak temperatures of 53–60 °C.
12.3.3.1 Advantages of MRgFUS
As a non-invasive, non-ionized MR-guided procedure with real time imaging and thermal feedback, MRgFUS has several advantages. The treatment can be monitored in real-time using MRI and MR-thermography. This allows for immediate confirmation of the targeting process. Thermal lesioning can be performed discretely and accurately, and can be evaluated immediately. Unlike stereotactic radiosurgery , MRgFUS does not use ionizing radiation and does not carry the risk of radiation-induced tumorigenesis. Because the MRgFUS procedure is non-invasive, there is no scalp incision, no burr hole, and no electrode penetrating the brain, unlike radiofrequency lesioning. Thus, MRgFUS reduces the risk of hemorrhagic complications and this non-invasive procedure also eliminates the risk of infectious complications. Compared with DBS treatment, there is no implanted hardware, no concern about interference with external sources of electromagnetic noise, no need for extensive follow-up for programming, and no need for periodic battery replacement. This represents a much simpler treatment plan for all patients, and will save hours of clinic time for DBS device management and replacement. Additionally, health care costs will be greatly reduced. It will be possible to re-treat a patient who develops a recurrence after other surgical treatments.
12.3.3.2 Potential Risks of MRgFUS
Although ultrasound techniques have advanced enough to overcome many former issues, skull heating may still be sufficient to damage bone and/or adjacent soft tissues. The sonication times should be calculated to keep skull temperatures below dangerous levels, and a minimum of 10 min assigned for skull cooling will provide time for the skull to return to normal temperatures before any additional heating takes place. Tissues along the path to the target (scalp, dura, arachnoid) and brain tissues adjacent to the target, also can become heated to the point where tissue damage or a burn might occur. This heating can be caused directly by improper treatment targeting, irregularities on the skin surface, treatment volumes of tissue that are too close to the skin or bone, or the conduction of sufficient heat to cause a burn at the surface. The presence of microcalcification in the brain tissue may create additional heating effects along the beam path. By utilizing the CT data, the ultrasound beam can be prevented from passing through these calcified areas.
Secondary hot-spot formation at bone-tissue and air-tissue interfaces is also a major concern, especially at the base of the skull. Pulkkinen et al. reported [44] that therapeutic ultrasound devices can produce potentially dangerous heating of the base of the skull. They determined safety limits, which apply for the thermal treatments operating at a frequency of 230 kHz.
Blood brain barrier (BBB) disruption, edema, swelling, and hemorrhage outside and remote from the targeted area also can occur. Theoretically, these events may be due to heating effects and/or to the pressure wave of the ultrasound beam. An increased rate of cerebral hemorrhage has been reported in stroke patients concomitantly treated with intravenous tissue plasminogen activator (tPA) [10].
High-field MR-induced vertigo is often observed in users of high-field (≥2 T) MRI scanners who experience disorientation or the subtle perception of movement when working close to or within the bore tube of the magnet, and this can also torment patients during the procedure. The patients will manifest symptoms such as nausea, vomiting, and dizziness. Although these symptoms may be temporary in most cases and disappear when the subject moves out of the magnet, the sensation of vertigo may accompany longer exposure. In our experiences of treating essential tremor using MRgFUS , half of the patients (5 among 11 patients) suffered from MR-induced vertigo [5]. Three main hypotheses were proposed for this effect: induced currents, which modulate the firing rate of the vestibular hair cells, magneto-hydrodynamics, and tissue magnetic susceptibility differences [19].
There is a risk associated with subject motion during a sonication or between sonications. This could cause a movement of the tissue relative to the planned treatment volume on the system, and in extreme cases could result in the treatment of a point outside the planned treatment volume. Because the skull functions as a defocusing lens, the phase correction map computed for the target spot, will also become ineffective if the subject moves.
If the CT and MR volumes are not well aligned with each other, the tuning of the ultrasonic elements will be suboptimal and distortion will make it difficult to achieve an exact volume of heating. The protocols for image fusion are well-established and will be visually confirmed by clinicians experienced in stereotactic targeting. Observation of the location, size and pattern of areas heated by the low-power sonication trials will also provide direct confirmation of the accuracy of the MRgFUS focus. MR thermography allows for the confirmation of accuracy before the process of therapeutic sonications begins.
12.3.3.3 Treatment Procedure for MRgFUS
The patient’s hair is carefully removed and the scalp examined for existing scars or any other lesions before the day of treatment. Just before applying head fixation, the patient’s hair is shaved again to prevent thermal injury to the hair. Overall steps in the treatment procedure steps are performed as follows (Fig. 12.1).
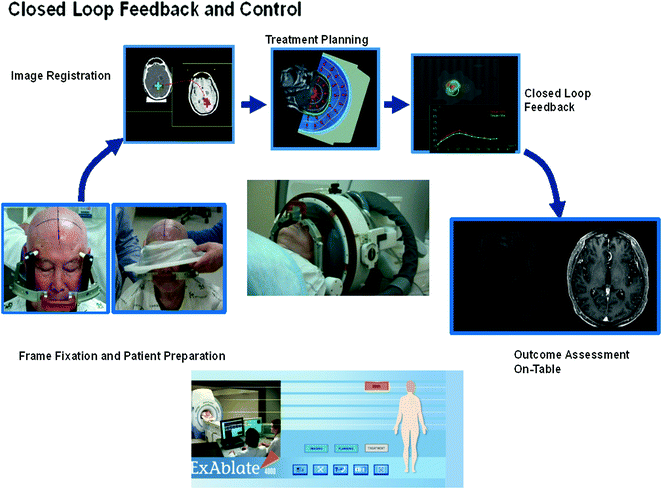
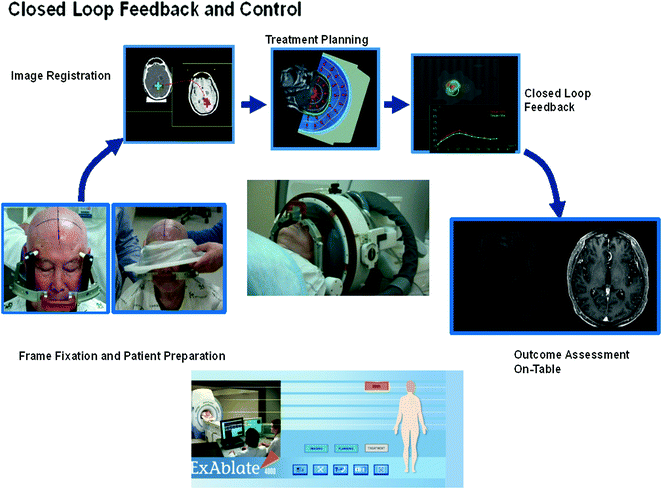
Fig. 12.1
Overall procedure of MRgFUS
1.
The patient’s head is placed in a MR-compatible stereotactic frame (similar to those used in stereotactic radiotherapy head fixation).
2.
The patient is positioned supine and headfirst on the MRgFUS therapy table.
3.
A hemispherical helmet containing the transducer elements is positioned on the patient’s head in the treatment position (this should be done according to measurements taken during the pre-operative imaging session).
4.
A rubber diaphragm is attached to the patient’s head and to the transducer to allow acoustic coupling between the ultrasound transducer and the scalp.
5.
The immobilization system is secured over the patient’s head to maintain a fixed position between the patient’s head and the ultrasonic transducer.
6.
A localizer scan (quick T1) and a T2 fast spin echo (T2-FSE) MR scan is obtained to allow further refinement of the transducer focal point with respect to the targeted zone.
7.
Then, the interface within the rubber diaphragm is completely filled with degassed water to avoid air bubbles between the transducer and the scalp (active circulation, degassing, and cooling of this water is continuously maintained throughout the procedure to avoid undesired heating of the scalp and skull).
8.
A series of MR images will be acquired to identify the target area, and plan the actual treatment:
a.
T2 Weighted imaging is examined along at least 2 axes: Axial and Sagittal.
b.
Other MR imaging series may also be acquired.
9.
The pre-treatment MRI and CT image datasets are registered to the T2 weighted MR images acquired in the previous steps. This image fusion of pre-operative MR assists in the accurate delineation of the target area and determination of a safe sonication pathway:
a.
The fusion of the CT data is required for the computation of phase correction values to correct for skull aberration, and identification of intracranial calcifications.
b.
Scars on the scalp are designated to ensure the ultrasound beam avoids these specific areas.
10.
The treating physician defines the treatment volume and plan. A commercial workstation automatically computes the number of sonications, and the phase and amplitude corrections (per sonication spot) necessary for the system to produce a focal spot at each of the desired locations.
11.
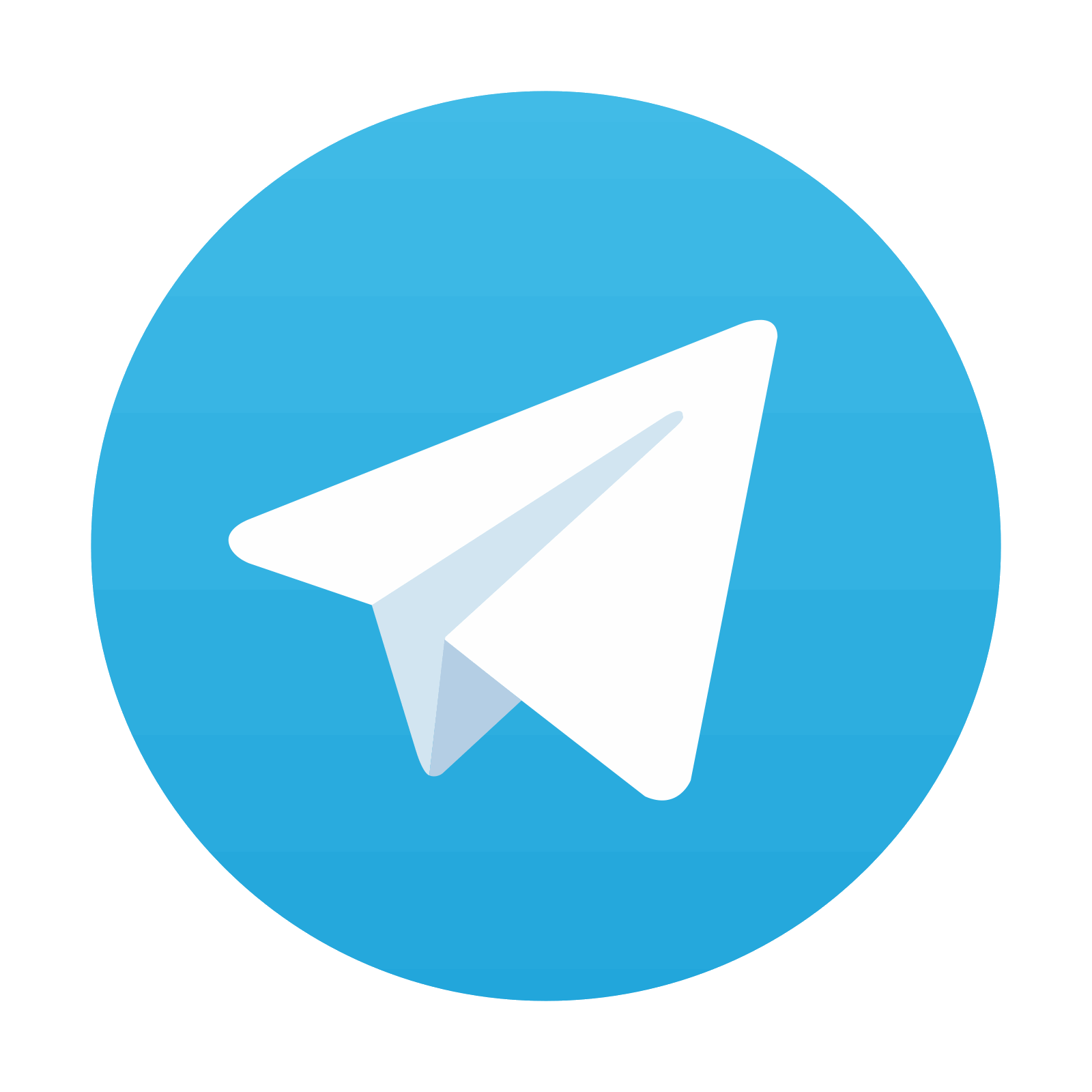
A central point in the targeted area is targeted by a low-dose sonication with a sub-lethal energy level to confirm the targeting accuracy on the MR images. Focal point position and/or transducer location are adjusted as necessary.
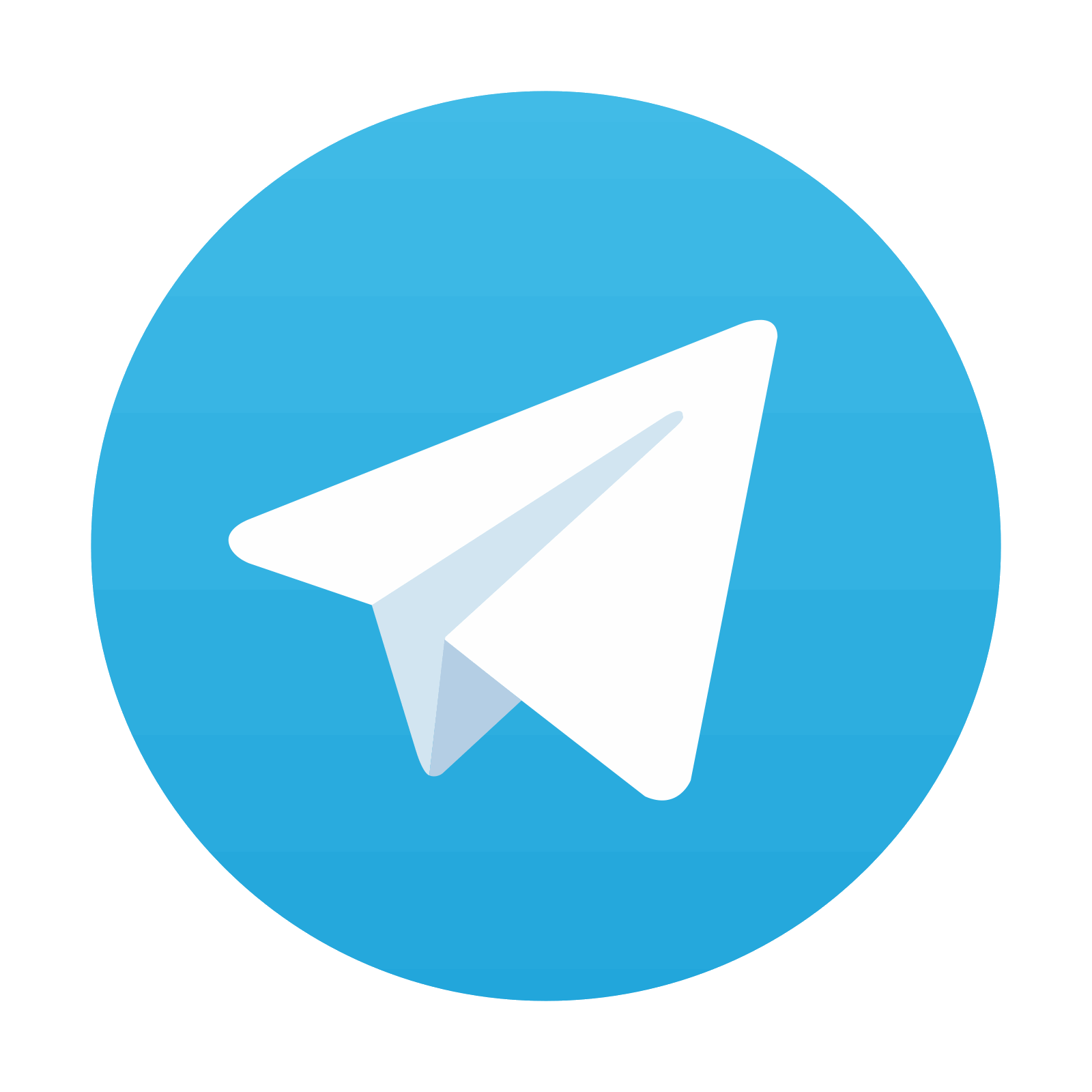
Stay updated, free articles. Join our Telegram channel
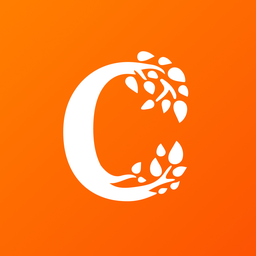
Full access? Get Clinical Tree
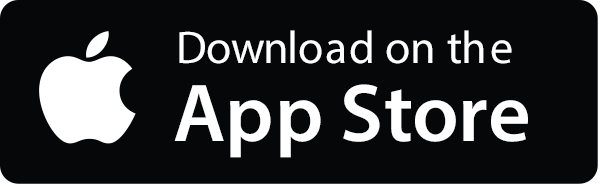
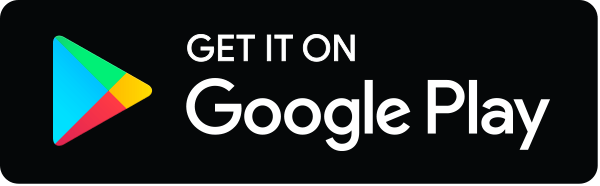
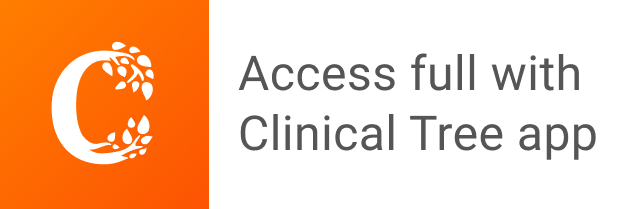