Functional Magnetic Resonance Imaging
E. T. Bullmore
J. Suckling
Introduction
Functional magnetic resonance imaging (fMRI) is a relatively new technique for measuring changes in cerebral blood flow. The first fMRI studies, showing functional activation of the occipital cortex by visual stimulation and activation of the motor cortex by finger movement, were published in the early 1990s.(1-3) In the years since then, fMRI has been used to investigate the physiological response to a wide variety of experimental procedures in both normal human subjects and diverse patient groups. In the next 10 years, fMRI will probably establish a role for itself in radiological and psychiatric practice; currently the clinical role of fMRI is limited to specialized applications such as assessment of hemispheric dominance prior to neurosurgery.(4)
The outstanding advantage of fMRI over alternative methods of imaging cerebral blood flow, such as positron emission tomography (PET) and single-photon emission computed tomography (SPECT), is that it does not involve exposure to radioactivity. This means that a single subject can safely be examined by fMRI on
many occasions, and that the ethical problems of examining patients are minimized. Functional MRI also has superior spatial resolution (in the order of millimetres) and temporal resolution (in the order of seconds) compared with PET and SPECT.
many occasions, and that the ethical problems of examining patients are minimized. Functional MRI also has superior spatial resolution (in the order of millimetres) and temporal resolution (in the order of seconds) compared with PET and SPECT.
In this chapter, we provide an introduction to technical issues relevant to fMRI data acquisition, study design, and analysis. An introduction to the basic physical principles of magnetization and nuclear magnetic resonance, and the technology, is given in Chapter 2.3.7.
Cerebral activation and blood-flow changes
Try closing your eyes and then opening them again. At the moment that you open your eyes, neurones in the occipital cortex that are specialized for the perception of visual stimuli will show a sudden and dramatic increase in their rate of discharge. There is a short delay (approximately 100 ms) between the stimulus and neural response owing to the propagation of electrical activity from the retina via the optic nerves and tracts to the visual cortex. Later, some 3 to 8 s after stimulus onset, there will be an accompanying change in the local blood supply to the stimulated area of cortex. Blood flow increases without a commensurate increase in oxygen uptake by the visual cortex, leading to a local increase in the ratio of oxygenated to deoxygenated forms of haemoglobin.
The linkage between neural activity and regional cerebral blood flow, sometimes called neurovascular coupling, has been known since Roy and Sherrington first reported ‘changes in blood supply in accordance with local variations of functional activity’ in 1894. However, the biophysical and biochemical mechanisms for neurovascular coupling are complex and not yet completely defined in detail.(7)
Endogenous contrast agents
The fact that neural activity is linked to local blood flow provides the opportunity for functional MRI. The most common, and non-invasive, approach exploits the paramagnetic properties of iron in deoxygenated haemoglobin as an endogenous contrast agent. Neural activity causes a local reduction in the ratio of deoxygenated to oxygenated haemoglobin, so that the paramagnetic effects of deoxyhaemoglobin are ‘diluted’. Since apparent spin-spin relaxation or dephasing is accelerated by microscopic inhomogeneities in the magnetic field due to the presence of paramagnetic contrast agents, the net effect of diluting deoxyhaemoglobin will be to prolong T*2 times in areas of the brain that receive an increased blood flow as a consequence of neural activity. The haemodynamic effect on spin-spin relaxation can be measured by a T*2-weighted signal change (of 3 per cent or less) which is blood oxygen level dependent (BOLD).
Imaging sequences for fMRI
Several different pulse sequences can be used to collect MRI data that are sensitive to functionally determined changes in signal strength. Here we will concentrate on gradient echo sequences which, combined with special techniques for very rapid data acquisition, are most widely used to date for fMRI. However, spin echo sequences can also be used for functional MRI data acquisition, and gradient echo sequences can be used for structural MRI.
Gradient echo sequence
The basic principle is similar to spin echo imaging. An initial excitation pulse of radiofrequency energy is supplied at the Larmor frequency to the brain in the presence of a powerful static magnetic field. Protons are excited to a state characterized by increased transverse magnetization and a coherent phase of precession around the axis of the external field. Immediately the radiofrequency pulse has ceased, protons begin to relax back to their equilibrium state of maximum longitudinal magnetization and random phase of precession, emitting a radiofrequency signal by free induction decay.
In gradient echo imaging, the process of spin-spin relaxation (dephasing) is first accelerated by briefly applying a gradient to the magnetic field shortly after the excitation pulse. Then, at some time (TE/2) after the excitation pulse, a second gradient is applied to reverse the process of dephasing, causing rephasing and a signal maximum or echo some time (TE) after excitation. The sequence is repetitively applied with a constant time interval between consecutive excitations (TR).
The objective is to manipulate spin-spin relaxation by brief perturbations of the external magnetic field rather than by supplying additional pulses of radiofrequency energy as in spin echo imaging. Frequency- and phase-encoding gradients are applied to locate the sources of signal in three-dimensional space (see Chapter 2.3.7).
One advantage of gradient echo imaging is that TE and TR can both be shorter than in spin echo imaging, allowing an overall reduction in scanning time. However, if TR is short, spoiler gradients or radiofrequency pulses may be needed to ensure that the protons have returned to equilibrium before the next excitation pulse is supplied. The flip angle a induced by radiofrequency excitation can be adjusted to generate images weighted by different sources of tissue contrast. T1-weighted images are generated by radiofrequency pulses causing flip angles of the order of 10° to 20°. For functionally sensitive T2-weighted images, more radiofrequency energy must be supplied in the excitation pulse to give a flip angle approaching 90°.
Echoplanar imaging
The gradient echo sequence equivalent to a fast spin echo sequence is obtained by rapidly applying, or blipping, a series of rephasing gradients following the excitation pulse and dephasing gradient. Gradient blipping is done extremely rapidly (<1 ms), and up to 128 echoes can be generated from a single excitation. Clearly, the advantage of such echoplanar imaging is the speed of acquisition. Multislice images of the entire cortex, with slice thickness of only a few millimetres, can be acquired in 2 s or less. Such high-speed imaging is highly desirable for functional MRI, where we wish to detect physiologically determined changes in magnetic resonance signal with the best possible temporal resolution. However, the hardware required for rapid gradient blipping has only become widely available in the last few years.
Artefacts
The main sources of artefact in functional MRI are the same as for structural MRI (see Chapter 2.3.7).
Movement
Movement of the subject’s head during fMRI data acquisition is inevitable, and attempts to eliminate it by fixing the head in the scanner may paradoxically exacerbate the problem. The best approach of minimizing movement is to ensure that the subjects are not unduly anxious about the scanning procedure, that they understand clearly what they are being asked to do, and that they are comfortable in the scanner before data acquisition begins. Experiments should be designed so that they do not require the subject to move extensively; small finger movements required for button pressing do not generally cause severe head movement. However, even very small movements of the head (less than 1 mm) can cause significant artefacts in fMRI data.
Involuntary or physiological movements are mostly due to the cardiorespiratory cycle causing pulsation of the cerebrospinal fluid and vascular spaces. Therefore, these movements often occur at a higher frequency than the frequency of image volume acquisition, and are aliased into the signal as a low frequency confound.
Susceptibility
The susceptibility artefact is exaggerated by gradient echoplanar imaging, typically causing signal loss in inferior temporal and orbitofrontal brain regions close to bone or sinuses. The problem is further compounded if subjects are asked to speak during scanning, since slight deformations of the sinuses associated with overt articulation can cause changes in susceptibility artefact, which can mimic signal changes due to speech-related neural activity. If overt articulation is necessary to monitor the subject’s performance on the experimental task, then it is advisable to design the sequence so that images are not acquired while the subject is speaking.
Hardware
The hardware requirements for functional MRI include a superconducting magnet, a radiofrequency coil, computers, and a purposebuilt room, as described in Chapter 2.3.7.
Gradient coils
The essential extra prerequisite is gradient coils capable of very rapidly blipping the external magnetic field for echoplanar imaging. The gradients required are small compared with the external field (1 to 10 mT/m) but may need to be applied for less than 1 ms. The speed with which the gradient is switched on, the slew rate, is necessarily fast (up to 200 mT/m/s). Such rapidly changing gradients can cause eddy currents in the gradient coils, adversely affecting the homogeneity of the field. This problem is minimized by actively shielding the coils, which requires yet another set of coils.
Audiovisual equipment
It must be possible to present visual and auditory stimuli to subjects while they are lying with their heads in the bore of the magnet. Headphones are required to clearly present auditory stimuli in the presence of the loud background noise of gradient switching. Visual stimuli can be projected on to a screen, viewed through a periscope. The subject should have access to an alert button. In addition, it is generally useful for subjects to use a button-press device to indicate their response to cognitively demanding experimental tasks. These behavioural data will need to be monitored during scanning.
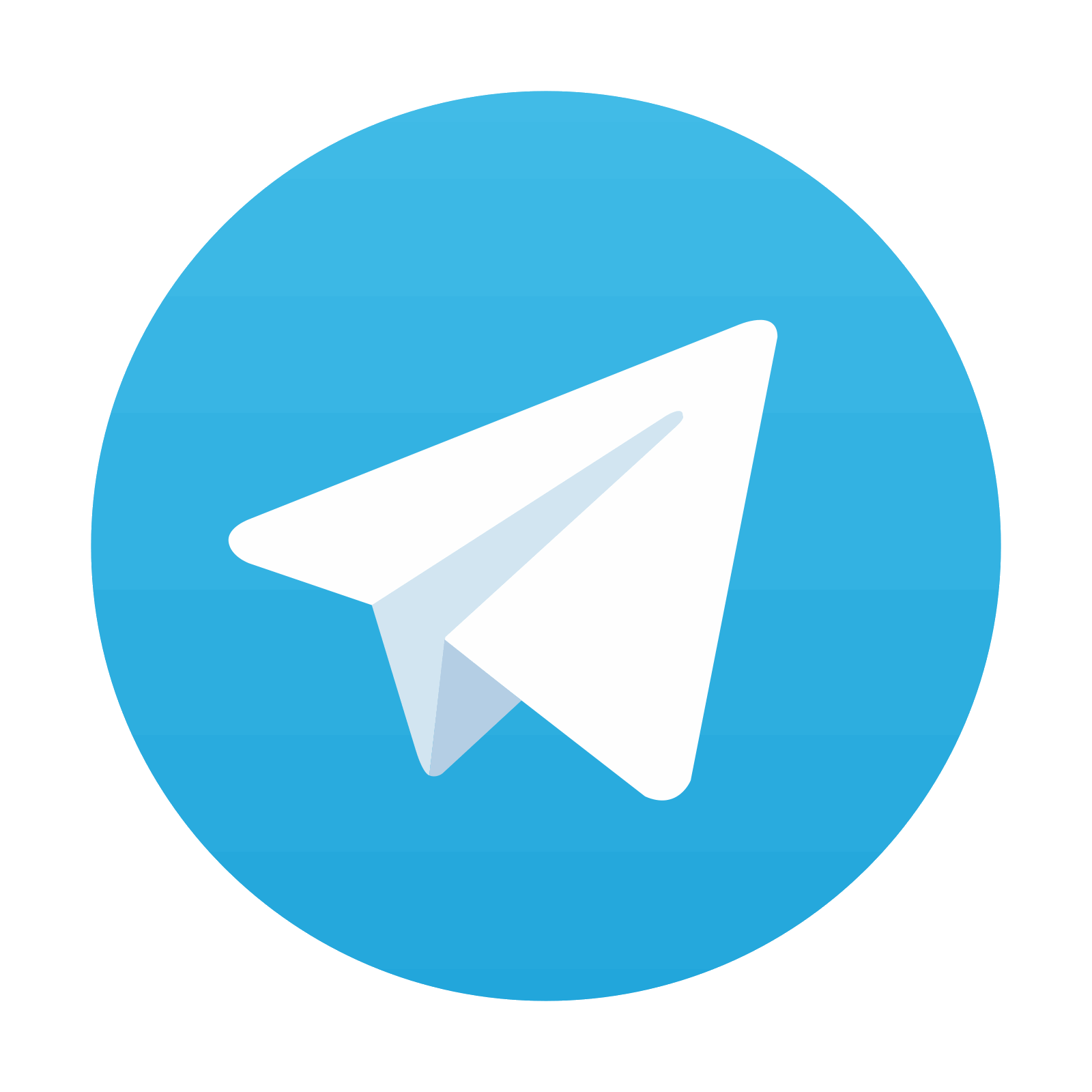
Stay updated, free articles. Join our Telegram channel
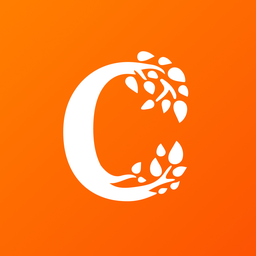
Full access? Get Clinical Tree
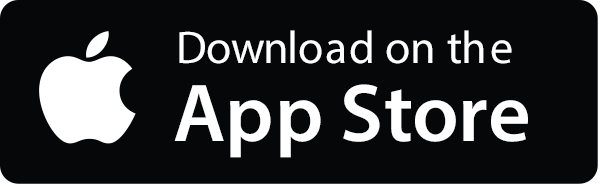
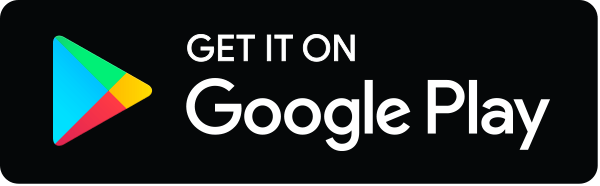