Tourette syndrome is defined by motor and vocal tics, yet our understanding of the pathophysiology of tics remains limited. Functional MRI (fMRI) can localize brain function related to the clinical phenomenology of tics. Here, we review extant fMRI studies examining brain activity during the premonitory urge, tic release, and tic suppression. Results are placed in the context of large-scale functional networks, given recent advancements in understanding the brain’s functional network organization. During tic-related phenomena, brain activity follows consistent patterns involving specific networks, largely centered around the cingulo-opercular action mode network. This network-level framework provides a novel avenue for targeted-treatment methods.
Key points
- •
Advancements in functional MRI methods have afforded the opportunity to localize tic-related brain activity in vivo in patients with Tourette syndrome.
- •
We review studies informing the neural correlates of the pre-tic time period, tic release, and tic suppression in the context of large-scale functional brain networks.
- •
Tic-related brain activity consistently involves particular functional brain networks, centering around the cingulo-opercular action mode network, which supports action planning, control of goal-directed behavior, and error detection.
- •
Placing the literature in a brain network framework highlights potential functional networks as targets for new investigative treatments.
Introduction
The past 25 y have seen a significant boom in our understanding of human brain function in neuropsychiatric disorders, including Tourette syndrome (TS). With the advent and increased use of brain imaging methods, researchers have been able to measure function across the whole brain in humans in vivo . In particular, functional MRI (fMRI) has become a standard method in neuroimaging research, in part, due to its noninvasive nature, superior spatial resolution, and increasing accessibility. Thus, investigators have taken advantage of the benefits of fMRI to study TS, broadening our understanding of function across the whole brain in this complex disorder.
fMRI is a powerful tool for measuring both evoked and intrinsic brain function with whole-brain coverage, which can be leveraged to map the functional neuroanatomy underlying TS. fMRI indirectly measures neuronal activity using the ratio of oxyhemoglobin to deoxyhemoglobin as a contrast mechanism, resulting in a blood-oxygen level-dependent (BOLD) signal. While fMRI cannot directly record neuronal or circuit function, it can chart and characterize the function of brain maps and systems. The BOLD signal changes when research participants in the MRI scanner perform a task (ie, task-fMRI), and different task conditions are contrasted to map brain regions with evoked activity during specific behavioral (eg, tics) or cognitive (eg, suppression) functions. At rest and in the absence of a specified task (ie, resting state fMRI), BOLD signal fluctuations can be measured over the time course of an fMRI scan. Functional connectivity is the correlation of this intrinsic activity between pairs of brain regions, and shows that distant, yet functionally related regions exhibit coordinated activity, even at rest. Thus, functional connectivity is used as a proxy for the functional relatedness of regions and is thought to reflect the statistical history of co-activation across the lifespan. Altogether, fMRI can be used to non-invasively interrogate specific motor, sensory, and cognitive responses, as well as brain-wide patterns of co-activation, all of which may be affected in TS.
Recent years have seen a shift toward examining brain function in neuropsychiatric disorders through a lens of large-scale brain systems. These functional systems, commonly referred to as “networks” in the cognitive neuroscience literature, comprise distinct, but functionally related brain regions. Canonical functional networks have been consistently identified using both task and resting state fMRI, as well as using varied analytical approaches. , These networks include “processing” networks that interface with the external world (somatomotor, visual, and auditory), “control” networks that direct attention and perform executive functions (dorsal attention, ventral attention, fronto-parietal, cingulo-opercular, and salience), and other association networks (default-mode, parietal memory, and reward). Functional network organization constrains learning and plasticity and can vary across individuals and in disease. Thus, placing the extant literature on the functional neuroanatomy of tics in TS within the framework of canonical functional networks may shed light on the intricate interactions of brain systems underlying symptoms.
While the clinical profile of TS can be complex, involving motor, sensory, and cognitive functions, the defining symptoms are motor and/or vocal tics. Thus, understanding how tics are generated in the brain is the key to understanding the major pathophysiology of the disorder. A prominent theory of tic generation was proposed by Mink, positing aberrant activity in cortico-striato-thalamo-cortical loops that leads to the disinhibition of unwanted motor plans, resulting in the production of tics. Consistent with this model, microstimulation and bicuculline (GABA-A antagonist) injections in the striatum elicit tic-like movements in rhesus macaques and rats. Post-mortem human studies have shown fewer parvalbumin positive inhibitory interneurons in the striatum of patients with TS, which could lead to hyperactivity of striatal neurons, consistent with a theory of aberrant activity. Additionally, smaller caudate and putamen volumes, as well as thinning in sensorimotor cortex, have been found in structural MRI studies of TS and were linked to more severe tics. While these data support the model, there are several phenomena that the model does not explain, such as the waxing and waning nature of tics, the effect of stress and attention on tics, and tics reducing or disappearing during sleep. A deeper understanding of the functional neuroanatomy of tics in the context of large-scale functional organization may inform a more complete model.
With fMRI, researchers can measure the BOLD response evoked when a patient exhibits a tic in real-time and map the brain regions recruited during tic generation and suppression. Several human fMRI studies have specifically investigated brain function during tics, identifying brain regions that are activated preceding tics, during tics, and during tic suppression. Here, we review those studies and consider the data within the context of large-scale functional brain networks. Fig. 1 depicts previously reported peak regions of activation from fMRI studies of tics overlaid onto canonical functional networks. Taken together, we propose a framework for understanding tics that encompasses multiple brain systems.

Tic-related brain activity
Studying tic-related brain activity with fMRI is not trivial. Experimenters must accurately identify the timing of tics while the participant lies inside the scanner. In most cases, tics are identified with audiovisual recordings taken of the participant during the scan, which then must be synced to the fMRI data. Video recording setups include video cameras positioned outside the scanner room, viewing the participant’s face and/or body through a window, or magnetic resonance (MR)-compatible cameras that can be placed inside the scan room or scanner bore. Audio recordings that are sensitive enough to detect vocal tics over the noise the scanner must be acquired using an MR-compatible microphone. At many institutions, MR-compatible recording equipment is not standardly provided by the facility and can be very expensive. In addition, the recordings of tics need to be manually coded to note the precise timing of tics, requiring trained raters and a significant time investment. Thus, audiovisual recording of tics during scans requires additional resources compared to typical neuroimaging research. If a research group does not have these resources, other creative approaches can be used. One study identified tics by localizing a tic reference region and correlating activity with that reference region (described more below). However, this approach comes with its own limitations.
Another challenge in measuring tic-related brain activity is that tics often involve movement. In fMRI data, head motion can cause signal artifacts, corrupting the data and confounding inferences. Not only do artifacts occur with tics that involve the head, but movement of other body parts will cause small head movements that are detectable during fMRI data acquisition. Therefore, comparing brain activity during the time in which a tic occurs to the time when a tic does not occur could simply be a comparison of more movement to less movement. Of course, not all tics cause head motion that affects the scan data, but it is not always predictable prior to scanning, which tics will have a significantly negative impact on the data quality. Thus, it is imperative that researchers in this field carefully consider the effects of movement, accurately measure head motion during scans, and use all experimental and data processing tools available to account for this issue.
When measuring brain activity during an event (here, tic), a minimum number of instances of the event must occur to obtain sufficient power to robustly detect an event-related change in the BOLD signal. Within a given participant in a typical task fMRI study, 25 events per condition yields stable activation maps. Therefore, to achieve sufficient power to detect tic-related activity during a single fMRI scan, one would need to record at least 25 tics during the scan, which often ranges from 5 to 20 min. Relying on the spontaneous occurrence of tics in the scanner, therefore, may not always result in enough tics per scan. Moreover, in order to have a sufficient number of events per scan, all types of tics (eg, eye blinks, throat clearing) are typically grouped together to measure all tic-related brain activity, even though patients with TS exhibit multiple types of tics that affect different parts of the body. Hence, when reviewing the literature on tic-related brain activity, one must be mindful of these challenges and limitations and how they are addressed in the experimental design.
The first human study of tic-related brain activity used PET in 6 participants with TS, setting the stage for imaging brain function during tics in human patients. This study first established specific cortical and subcortical brain regions with increased tic-related activity, including the supplementary motor area (SMA), anterior cingulate cortex (ACC), premotor cortex, insula, primary motor cortex (M1), primary somatosensory cortex (S1), prefrontal cortex, parietal regions, caudate, putamen, and thalamus. A later PET study found overlapping results during tic release in the insula, putamen, and thalamus, as well as increased activity in the cerebellum. The first use of fMRI to investigate tic-related brain activity was a case study of a 15 y old boy with coprolalia, and results showed increased BOLD signal associated with tics in the cingulate gyrus, caudate, and several parietal regions, demonstrating some consistency with the PET studies. Although these studies were limited by small samples and modalities with poorer resolution than current methods, they established sets of brain regions, both cortical and subcortical, that are involved in tics.
Pre-tic Brain Activity
Several studies have taken advantage of the increased temporal resolution of fMRI in comparison to PET to separately examine the time period preceding tics from the time period during tics. This approach potentially targets the clinical phenomena of the premonitory urge, the sensation of discomfort that builds up prior to a tic. The release of the tic then relieves the discomfort from the urge. By examining brain activity during the time period prior to a tic separately from the time period during a tic, we can better understand the mechanisms involved in these clinical phenomena. Three fMRI studies examined brain activity prior to a tic in order to capture the time period associated with the premonitory urge. Two of these studies analyzed tics that were manually coded as events akin to a task-fMRI design of evoked behavior, specifically identifying brain regions with increased activity 1 to 2 sec prior to a tic. , The other study relied on participant reports of tics (button presses) to determine timing and identify brain regions with increased activity before, as well as during and after tics. A fourth study aimed to engage urge-related activity by instructing participants to imagine situations that trigger their tics and rate their distress levels during those imagined scenarios. Brain regions were identified in which increased activity during imagined, urge-inducing situations was associated with urge-related distress.
In all the 4 studies, the SMA showed a significant effect (ie, increased activity prior to tics in the first 3 studies, and a relationship with urge-related distress in the fourth). As the SMA is involved in motor planning and initiation and electrical stimulation to this region elicits the urge to move, aberrant SMA function could reasonably result in the urge to tic. The convergence of this finding with a sensible mechanistic explanation led to a focus on the SMA as a locus of tic generation and a possible target of treatment in TS. In fact, a randomized controlled study of neurofeedback of SMA activity demonstrated a significant decrease in tic severity compared to a sham control. Additionally, the SMA is a major cortical site targeted in current investigations of transcranial magnetic stimulation (TMS) as a treatment for TS. ,
In addition to the SMA, 2 of the task-fMRI-like studies of the pre-tic time period , and the study using imagined urge scenarios found consistent activity in several other brain regions, including the parietal operculum (which includes supramarginal gyrus), insula, and thalamus. Given the parsimonious explanation of the SMA as a driver of tics, the involvement of these other regions in the premonitory urge was somewhat overlooked. The consistencies across studies in these regions, however, suggest that there is a more complex interaction of brain regions associated with tic generation beyond just the SMA, sparking our interest in considering neuroimaging findings in the context of large-scale brain networks.
The SMA, parietal operculum, insula, and thalamus are all parts of the cingulo-opercular network, recently termed the action-mode network (henceforth referred to as the CON/AMN). Fig. 1 A displays centers of pre-tic activation for those studies specifically measuring the pre-tic time period overlaid onto the canonical CON/AMN. This network was first described as a top-down executive control system, producing signals at the initiation of cognitive tasks, during sustained maintenance of goal-directed behaviors, and when detecting errors. , Additional work has revealed a role in pain processing, ambiguity, motor plasticity, and action planning and preparation. Taken together, the evidence supports the implication of the CON/AMN as controlling the brain’s action-mode, the dipole of the brain’s default-mode (for further discussion, see ). This recent understanding sheds light on the studies reviewed here that show increased activity in core regions of the CON/AMN during the pre-tic period, and may indicate engagement of the brain’s action mode during the premonitory urge. Such engagement might reflect aberrant action preparation or the detection of an errant motor plan, resulting in a feeling of discomfort or urge to tic.
Brain Activity During Tic Release
A few fMRI studies have specifically examined the time period during the tic itself. Two of the task-fMRI-like studies discussed earlier also examined BOLD activity at tic onset. , Both found increased activity in somatomotor regions (M1, S1, and thalamus), as would be expected with tics. Bohlhalter and colleagues also reported increased activity in the superior parietal lobule and dorsolateral prefrontal cortex, while Neuner and colleagues also reported increased activity in the central operculum (which includes the insula and supramarginal gyrus). These findings overlap markedly with the results of the PET studies discussed earlier. However, increased activity in primary somatomotor regions would be expected with non-tic related movements as well, and hence, it is difficult to assess if this activation was specific to tics.
One approach that eliminates motion from the experimental design is the use of imagined scenarios, as presented in Zapparoli and colleagues. Participants were instructed to imagine performing their tic and were then asked to rate their perceived relief upon having performed the tic. Brain activity during imagery of performing a tic correlated with the participants’ perceived relief in the prefrontal cortex, insula, superior temporal gyrus, parieto-occipital regions, thalamus, pallidum, and putamen. The overlap with the task-fMRI-like studies related to tic release in the prefrontal cortex, insula, and thalamus indicates that activity in these regions may be independent of the actual movement associated with ticcing. It is important to note, however, that tics are suggestible and thinking or talking about one’s tics often elicits performing the tics. It is unclear whether or not the participants in this study did indeed perform their tics when imagining them; as such information was not reported.
Another experimental design approach to control for movement is to compare tics to voluntary movements. Wang and colleagues employed such a design, instructing participants with TS to either tic naturally or perform voluntary tics. Thus, both conditions included movement, but the origin of the movement was more controlled. In addition, a control group was instructed to perform either self-paced or cue-paced imitations of a tic for comparison analyses. In the TS group, increased activity for spontaneous versus voluntary tics was found in S1, posterior parietal cortex, putamen, and amygdala/hippocampus. When comparing spontaneous tics in TS to self-paced imitated tics in controls, increased activity was observed in primary sensorimotor regions, premotor regions, prefrontal cortex, posterior parietal cortex, and subcortical regions (including putamen and thalamus), as well as decreased activity in the ACC and caudate nucleus. These results indicate that increased activity in sensorimotor regions is not simply due to movement, but does differentiate tics from non-tic movements. A major limitation of this study, however, is that performance of voluntary tics in patients with TS can lead to sensory urges and performance of actual tics due to the suggestibility of tics. Therefore, it can be difficult to disentangle if the voluntary tic condition is truly distinct from the spontaneous tic condition. In fact, Bohlatler and colleagues included a tic imitation condition, but could not analyze the data with sufficient power for several reasons. For one, they found it difficult to distinguish voluntary versus actual tics upon video analysis. Also, some participants moved their head much more during tic imitation, creating an additional confound for this type of experimental design.
Across studies examining brain activity during tics, there was consistent activation in somatomotor regions, including M1, S1, thalamus, and putamen at tic onset ( Fig. 1 B). Investigations of large-scale functional network organization in the human brain have demonstrated somatomotor networks that do not separate by anatomic boundaries of somatosensory and motor functions across the central sulcus. Rather, functional divisions of somatomotor networks follow somatotopy with each division including both S1 and M1. One commonly used functional network convention describes 2 somatomotor networks, one encompassing ventral S1/M1, referred to as the somatomotor face/mouth network, and another encompassing dorsal and medial S1/M1, referred to as the somatomotor hand network. , Activation was found across both somatomotor functional networks in the studies of tic release , and remained when tics were contrasted with both voluntary movements and with control participants’ imitated tics. These findings support the perhaps unsurprising assumption of tics engaging both functionally defined somatomotor systems.
Interestingly, activation during tic release was also reported in the superior parietal lobule, supramarginal gyrus, and prefrontal cortex, which are parts of the dorsal attention network (DAN), and in several CON/AMN regions (see Fig. 1 B). The DAN is involved in top-down goal directed attention and is important for directing attentional resources. Individuals with parietal lesions in the DAN have difficulties attending to external and internal stimuli in specific spatial locations. Activation of the DAN during tics might suggest that the release of tics engages attentional resources. Indeed, tics are influenced by attention, as talking about one’s tics can exacerbate tic activity, while engaging attention elsewhere often reduces the production of tics. Activation of brain regions within the CON/AMN may be due to continued engagement of this network from the pre-tic, premonitory urge phase that persists into the time of actual tic release. Consistent with this idea, the relief associated with tic release does not necessarily occur right at tic onset; rather a tic may need to be performed completely or several times to achieve a “just right” feeling. Thus, the continued engagement of the CON/AMN may reflect the urge that is not quite relieved until the tic is satisfactorily completed.
Brain Activity During Tic Suppression
Tics have been described as “semivoluntary” and “unvoluntary” because patients often have the ability to suppress tics to an extent, at least for a short period of time. Patients will attempt to and will learn to inhibit their tics in various contexts (eg, social situations, school), resulting in tic suppression becoming a part of everyday life. Understanding how brain function supports this phenomenon would inform theories of how tics can be controlled, how this control develops with age, and why some people are better able to suppress tics than others.
One of the first fMRI studies of TS investigated tic suppression using a simple block design. Brain activity was contrasted during blocks of time when patients were instructed to suppress tics to blocks of time when patients were instructed to tic freely. This comparison showed increased activity during tic suppression in the superior and middle temporal gyrus, ACC, frontal cortex, inferior occipital cortex, and caudate nucleus. Reduced activity during suppression, which is mathematically equivalent to increased activity during the tic freely condition, was found in sensorimotor cortex, posterior cingulate cortex, cuneus, inferior parietal cortex, hippocampus, thalamus, putamen, and pallidum; several of these regions are consistent with the findings of the tic release studies discussed earlier. A later study using a similar paradigm (tic suppression vs tic freely) measured functional connectivity, specifically computing regional inhomogeneity, which is a measure of local connectivity. This study found increased regional inhomogeneity associated with tic suppression selectively in the left inferior frontal gyrus.
One limitation of a tic suppression paradigm is the lack of a control group. In order to make comparisons to a control group, several investigators have taken advantage of a motor action that everyone experiences and has similar properties to tics: blinks. Blinking tics (eg, hard blinks, multiple blinks in succession) are some of the most common tics in TS. Blinks can also be described as semivoluntary, since they can be suppressed for a period of time, during which the urge to blink builds until a blink is finally released. Therefore, blink suppression can be studied in a similar way to tic suppression and may engage similar neural circuitry. Van der Salm and colleagues designed an fMRI study in which they compared tic suppression in patients with TS to blink suppression in control participants. The contrast of tic suppression versus tic freely conditions in the TS group exhibited increased activity in the superior and middle temporal gyrus, superior frontal gyrus, prefrontal cortex, inferior parietal cortex, and inferior occipital cortex, consistent with the earlier findings of Peterson and colleagues. With the addition of the control group, they were able to compare tic suppression in TS to blink suppression in controls, allowing them to distinguish brain activity involved in suppression specific to tics, not other motor behavior. Results showed increased activity in the prefrontal cortex, superior frontal gyrus, ACC, and premotor cortex associated with tic suppression. These results provide additional evidence that several frontal regions are involved in suppressing tics, and that this involvement is beyond that of typical suppression of other motor behavior (ie, blinks).
One could argue that comparing tic suppression in TS to blink suppression in controls does not fully account for the differences between tics and blinks. Thus, there have been additional studies investigating blink suppression in both TS and controls. Hence, while tic suppression itself is not directly studied, the related ability to suppress blinks is tested in both groups. Two studies employing this design found increased activity in the superior temporal gyrus for blink suppression in TS compared to controls, with one of the studies finding additional activity in ACC, middle temporal gyrus, and superior frontal gyrus, and the other study finding additional activity in the supramarginal gyrus and midcingulate.
Across tic and blink suppression studies, there are consistent findings of activity in the middle and superior temporal gyrus and several frontal regions, including the ACC and prefrontal cortex. Fig. 1 C shows that these regions fall within the default-mode network (DMN) and the CON/AMN. The precise role of the DMN is not fully understood, but there is evidence that it supports internal, self-referential processing (eg, thinking about oneself), retrieving autobiographic memories, and monitoring social aspects of self. Interestingly, several resting state fMRI studies demonstrate altered DMN connectivity in TS (for a review, see ). Given its role in internal, self-referential processing, it has been posited that the DMN is involved in tic suppression by monitoring premonitory urge sensations. In addition to the DMN, tic/blink suppression also elicited activation in the CON/AMN. Given the posited role of the CON/AMN in the premonitory urge, this result is not unexpected, as the urge is likely present and builds up when attempting to inhibit tics. Thus, when suppressing tics, the DMN may be recruited to monitor the aberrant CON/AMN activity related to the premonitory urge.
Discussion
While previous neuroimaging work provided a valuable description of brain function in TS at the level of brain regions, considering these results in the context of whole-brain functional networks may yield a more complete understanding of tics and their associated clinical phenomena. Delineating the specific networks involved could facilitate interpretations that leverage the extensive work elucidating the functional properties of these networks. For instance, when frontal cortex activity in TS falls in regions belonging to the CON/AMN, we can consider such results in terms of the brain’s action mode, including functions such as motor planning and error signals. On the other hand, when the activity falls in regions belonging to the DMN, we can consider such results in terms of self-monitoring and self-referential processing. Though speculative, we can leverage our growing knowledge of the functional roles of large-scale brain networks to help inform our understanding of clinical symptoms.
Interestingly, tic release and tic suppression were associated with increased activity in multiple functional brain networks (eg, CON/AMN and DMN activation during tic suppression). Given years of experience living with tics, co-activation of multiple networks may lead to changes in the coordination of these systems. Functional connectivity, one measure of the coordination of brain systems, is thought to reflect the statistic history of co-activation over time. Indeed, we have demonstrated that atypical patterns of functional connectivity in TS differ between children and adults. This difference in development is consistent with the idea that tic-related brain activity contributes to changes in large-scale brain organization over time in TS.
The canonical brain networks considered here have predominantly been delineated in the cerebral cortex. However, subcortical regions (eg, thalamus, striatum) are certainly involved in TS, and the studies reviewed here show tic-related activity in these regions. Thus, it would be a mistake to ignore how the function of subcortical regions fits into the previously established large-scale functional networks. It is well-established that there is intricate connectivity between the cortex and subcortex, such that parallel yet integrated cortico-striato-thalamo-cortical loops exist to support various functions (eg, motor, cognitive). Functional connectivity investigations show that subregions of subcortical structures are functionally connected with cortical networks in complex but systematic ways. Unfortunately, the subcortical clusters of activation in the studies reviewed here span large areas and are referred to in their entirety (eg, thalamus), making it difficult to delineate the specific subregions and functional networks associated. Thus, the role of subcortical activity in the clinical phenomena of tics should be targeted more precisely in future investigations. For example, a subregion of the ventrolateral thalamus has been described as an integration zone, in which somatomotor networks and the CON/AMN converge. , Given the relevance of these networks for tics, this thalamic region is an intriguing candidate for targeted study.
Considering symptom-related brain function in the context of large-scale functional networks can influence our thinking about targets for treatment, particularly those that target specific brain regions, such as TMS, transcranial direct current stimulation, or deep brain stimulation (DBS). As mentioned earlier, the SMA is a current investigative target of TMS to treat tics. , The SMA resides on the medial wall of the frontal cortex, and is not always an easy target to selectively stimulate with the TMS coil placed over the head. Since the SMA is part of the CON/AMN, a potential alternative is to stimulate other regions in that network, especially given that several key regions of the CON/AMN show increased tic-related activity. Regions of the CON/AMN on the lateral surface of the cortex may be more easily accessible to the TMS coil and may affect the function of the network in a similar manner.
DBS has also shown efficacy in treating TS, specifically targeting subcortical regions. Since DBS requires invasive brain surgery, it is only used when nonsurgical alternatives fail. Unfortunately, subcortical structures are too deep in the brain to be targeted precisely with less invasive methods, like TMS. However, by mapping the cortical and subcortical components of functional networks, we could indirectly target the subcortical region of interest by accessing a cortical node of the network. There is evidence in obsessive-compulsive disorder (OCD) that DBS has effects at a network level, which is promising for targeting other parts of the network with noninvasive methods. Thus, rather than focusing treatments on targeting a single region, considering the effects of the network as a whole may open possibilities for alternative more tolerable treatments and treatment locations.
Summary
Here we reviewed fMRI studies of tic-related brain activity and discuss the results in the context of large-scale functional networks. This review of the literature demonstrates that engagement of brain regions belonging to the same functional networks follows a consistent pattern for each of 3 key clinical phenomena associated with tics: the premonitory urge, tic release, and tic suppression. The time preceding a tic, during which the premonitory urge occurs, engages the CON/AMN, a network supporting the brain’s action mode. Tic release continues to engage the CON/AMN, while also recruiting somatomotor networks and the DAN, a network involved in goal-directed attention. Tic suppression also engages the CON/AMN, likely due to the building premonitory urge when attempting to inhibit tics, and the DMN, which is involved in self-referential processing and has been proposed to monitor the urge. Hence, we present a framework for the functional neuroanatomy of tics with which we can leverage our knowledge of functional brain networks to deepen our understanding of tics and generate hypotheses for future research.
Clinics care points
- •
A better understanding of the pathophysiology of tics would help to advance identification of new targeted-treatment options for patients with TS.
- •
Neuroimaging studies have implicated specific brain regions in the clinical phenomenology of tics, such as activity in the SMA underlying the premonitory urge. Investigative treatment methods for TS, such as TMS and neurofeedback, have therefore focused on targeting the SMA. However, placing the results in the context of large-scale functional brain networks, rather than single brain regions, reveals engagement of multiple nodes of these networks. Hence, these investigative treatments should consider the entire network, rather than a single node.
- •
Across studies of the pre-tic premonitory urge, tic release, and tic suppression, increased brain activity occurred in multiple regions of the CON/AMN, including the SMA. Thus, the CON/AMN as a whole, or multiple nodes of this network, may be particularly useful in the identification of new treatment targets and approaches, as well as in tracking clinical outcomes.
- •
A network approach to treatment also opens possibilities for moving from invasive neurostimulation (eg, DBS), which is necessary for targeting deep subcortical structures, to non-invasive methods (eg, TMS), in which stimulating cortical regions may propagate through the entire network to affect those subcortical structures.
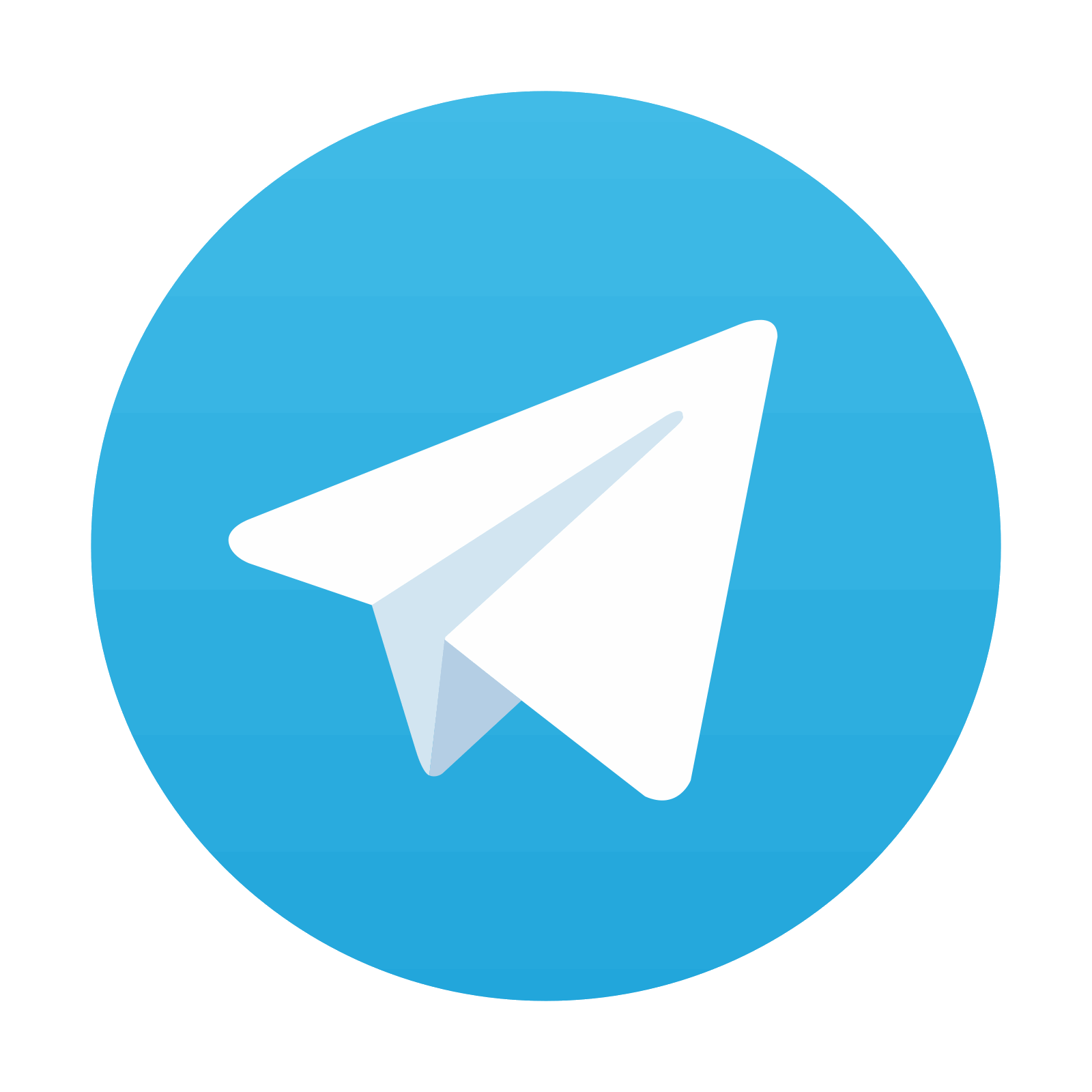
Stay updated, free articles. Join our Telegram channel
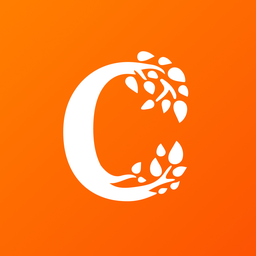
Full access? Get Clinical Tree
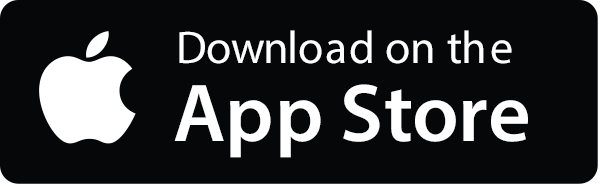
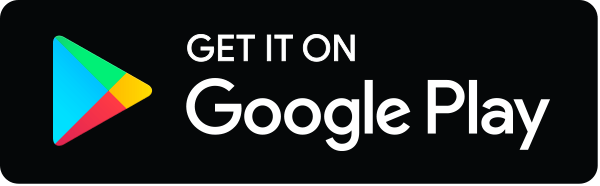
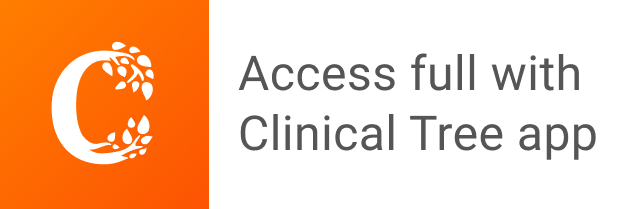