Functional Neuroimaging in Pediatric Populations
Daniel J. Simmonds
Stewart H. Mostofsky
The advent of functional magnetic resonance imaging (fMRI) has allowed us to see the living brain “at work.” fMRI studies in children have been an important step in understanding neural circuits involved in motor, sensory, cognitive, and behavioral functions that are key to normal development, as well as differences in those functions associated with pediatric clinical populations. This chapter is divided into three sections. First, it presents the general principles of fMRI, as well as its advantages over different neuroimaging techniques and its limitations, including specific caveats of the application of fMRI to pediatric populations. Second, it focuses on the specific applications of fMRI to attention deficit hyperactivity disorder (ADHD) as an example of a population extensively studied using fMRI, discussing behavioral findings in ADHD and how fMRI findings in the disorder complement understanding of its clinical profile. Finally, the chapter concludes with how the strategies for using fMRI to study ADHD can be extended to other clinical populations and how these data will inform the neural bases both of clinical disorders and of typical development.
Functional Magnetic Resonance Imaging
Magnetic resonance imaging uses a series of radio frequency (RF) pulses in combination with time-varying magnetic fields in order to image biological tissue. Inside the MRI scanner, hydrogen atoms within a person’s body align with the static magnetic field. Electromagnetic RF pulses delivered by the scanner excite the hydrogen nuclei and disrupt their alignment with the magnetic field. After excitation, the nuclei undergo both longitudinal relaxation, in which the nuclei realign with the static magnetic field, that is, the net magnetization recovers along the longitudinal (parallel) axis, and transverse relaxation, in which the net magnetization in the transverse (perpendicular) plane decreases as the nuclei lose their phase coherence. The longitudinal relaxation process is called T1 recovery and is what contributes to anatomical T1-weighted images, whereas the transverse relaxation process leads to T2 decay, which contributes to anatomical T2-weighted images, and T2* decay. The latter is especially relevant to fMRI, as it forms the basis of blood-oxygenation-level dependent (BOLD) imaging (1).
MECHANISMS OF THE BLOOD-OXYGENATION-LEVEL-DEPENDENT RESPONSE
The basis for the BOLD contrast is the sensitivity of T2*-weighted images to local amounts of deoxygenated hemoglobin, which disrupt the static magnetic field in the scanner due to its strong paramagnetic properties. Deoxygenated hemoglobin changes in accordance with the metabolic demands of neurons; increases in neuronal activity result in an increase in metabolic demands, necessitating the delivery of oxygen to the active neurons. Hence, neuronal activity is reflected by a delayed increase in oxygenated hemoglobin and a decrease in deoxygenated hemoglobin. This “hemodynamic response,” which is equivalent to the BOLD contrast, generally peaks about 4 to 6 seconds after neuronal activity (Fig. 9.1) (2). By taking a number of BOLD-sensitive images over time, localized changes in deoxyhemoglobin concentration corresponding to a priori task-dependent models can be measured. For fMRI, these images are usually acquired using echo-planar imaging (EPI), which is sensitive to the BOLD contrast and allows the brain to be rapidly imaged in slices (3).
TASK DESIGN IN FUNCTIONAL MAGNETIC RESONANCE IMAGING
Given that the brain is never “at rest” and local metabolic demands are constantly changing, traditional fMRI studies typically rely on contrasting activation during the experimental task with a baseline condition, such as a rest period or a different task. This technique is called “cognitive subtraction,” whereby it is inferred that differences in the BOLD contrast, or “activation,” between the two conditions are due to cognitive processes present in the experimental condition but not in the baseline condition.
Early fMRI studies used a block design approach adapted from positron emission tomography (PET) studies, whereby individuals perform a task for a period of time, and activation is averaged across the whole block. Due to fMRI’s superior temporal resolution in comparison to PET, many investigators quickly moved to event-related designs, whereby activation due to a single event can be modeled. Early event-related fMRI studies used periodic designs in which events were separated by more than 15 seconds in order to allow the hemodynamic response to relax, so that the BOLD signal did not saturate with rapidly repeated events (4); however, these designs were inefficient and inconsistent with the psychodynamic properties of tasks used to examine behavior outside the scanner. More recently, designs began to incorporate “jittering,” in which events occur at irregular intervals, allowing for interevent intervals as low as 500 ms (5).
Recently, newer methods of analysis have emerged, such as functional connectivity, which permit analysis of functional brain activation in the absence of an experimental task. Such studies of “resting-state” brain activity are further discussed later in this chapter.
ADVANTAGES OF FUNCTIONAL MAGNETIC RESONANCE IMAGING
There are several advantages to using fMRI rather than other functional neuroimaging techniques. Perhaps the most convincing is that fMRI is noninvasive, unlike PET and single-photon emission computed tomography (SPECT), which require injection of radioactive contrast agents. Its noninvasive nature makes fMRI better suited for longitudinal studies and pediatric studies (6).
Another advantage of fMRI is its balance of spatial resolution and temporal resolution. Typical fMRI studies have a spatial resolution on the scale of 2 to 4 mm, allowing detection of BOLD contrast changes (activation) in relatively small brain structures, such as the amygdala. On the temporal scale, whole-brain image volumes in fMRI are typically taken every 2 to 3 seconds, which is a high enough resolution to sample the hemodynamic response to single events. In contrast, PET has a temporal resolution ranging from 90 seconds for 15O to 30 to 40 minutes for 18F, due to its low signal-to-noise ratio (2). The temporal constraints of PET limit its use to studies with block designs, in contrast with the rapid event-related capabilities of fMRI.
LIMITATIONS OF FUNCTIONAL MAGNETIC RESONANCE IMAGING
Although fMRI has the best spatial resolution of the current human neuroimaging techniques and is capable of imaging brain function on a millimeter scale, it is unable to capture activity on a submillimeter scale, as manifested in individual neurons, axons, and synapses. In addition, the temporal resolution of fMRI, on the scale of seconds, cannot detect electrophysiological changes, which are on the scale of milliseconds; this is possible in humans only by using techniques such as electroencephalography (EEG), which has poor spatial resolution, and magnetoencephalography (MEG), which can detect activity only from neurons that are oriented perpendicular to the scalp.
An important consideration in fMRI is that the changes in signal are on the scale of 1% to 3%, depending on the strength of the scanner. To achieve enough power to detect a signal, a large number of trials must be averaged, which results in longer tasks and longer time spent in the scanner. This also means that sample sizes must be adequate to achieve enough power on a group level.
Another limitation in fMRI, particularly with EPI, is the presence of magnetic susceptibility artifacts that occur at air-tissue interfaces, most notably in the orbitofrontal cortex, adjacent to the nasal sinuses. The artifacts are due to magnetic field inhomogeneities, causing signal loss and compromised images of these regions. However, recently developed
scanning techniques show promise for minimizing these artifacts (7,8). Other scanner artifacts include those caused by metals with ferromagnetic material entering the scanner. Although some metal, such as dental fillings, do not induce artifact, implants with ferromagnetic material may preclude individuals from being scanned.
scanning techniques show promise for minimizing these artifacts (7,8). Other scanner artifacts include those caused by metals with ferromagnetic material entering the scanner. Although some metal, such as dental fillings, do not induce artifact, implants with ferromagnetic material may preclude individuals from being scanned.
Head motion during scanning, which tends to occur more in children, is another factor that compromises image quality. Given the fine spatial resolution of fMRI, even slight movements can cause the same brain region to be measured at different locations in space and alter the images. The need to restrict movement limits the use of tasks requiring verbal responses because oral movements are likely to result in changes in head position. Various software packages that can correct for motion artifacts are available (9); however, if the motion is correlated with the task (e.g., face imitation task in which subjects are required to mimic facial movements seen on a screen), it may be difficult to correct for motion without removing effects of interest. Approaches for minimizing motion in pediatric populations are discussed in the following section.
CAVEATS OF PEDIATRIC FUNCTIONAL MAGNETIC RESONANCE IMAGING
Using fMRI in pediatric populations poses unique methodological challenges. As mentioned in the previous section, one major limitation of fMRI is its susceptibility to even small amounts of motion, necessitating the subject to remain very still during scanning. This can be difficult for children, especially those with clinical diagnoses such as ADHD. To reduce motion during scanning, several methods of restraint are available, including bite bars, forehead and chin straps, and pillow/foam padding; however, many of these restraints will be unappealing to children. As such, minimizing these restraints will enhance tolerability.
Claustrophobia is another common challenge, given the relatively narrow and small space within the scanner. The head coil that is necessary for scanning can further compound this feeling. In addition, the scanner emits loud noises, which can be distressing to children who are sensitive to such stimuli, such as those with autism. All of these factors underlie the importance of having children participate in a “mock scan,” which simulates the environment and noises of the actual scanner. First, the mock scanner gives children a chance to acclimate to the scanner environment, which can help to reduce anxiety associated with entering the actual scanner for testing (10). Second, behavior management approaches, involving feedback with use of reinforcers, can be used to train children to lie still over the course of the mock scan, which subsequently helps to reduce motion during the actual scan (11). Finally, the mock scanner gives children the opportunity to ask questions before, rather than during, the time when the actual scanning takes place.
Another critical issue for pediatric fMRI is distractibility and boredom. Children frequently tend toward self-entertainment such as humming and singing, especially in boring or tedious environments, and hence need periodic reminders of which behaviors are unacceptable in the scanner, although training in the mock scanner may help alleviate this. One of the most common causes of these unacceptable behaviors is using a task that is developmentally inappropriate, that is, one that is either too difficult or too easy. Suitable tasks are those in which the instructions are explained simply and clearly so that the child understands and can comply, cognitive demands are commensurate with the child’s level, and duration is minimized in order to avoid the risk of the child’s not cooperating late in the session.
There are aspects of fMRI image processing for which special considerations exist with regard to the study of children. As each individual’s brain is unique, both in size and in morphology, all individual brains need to undergo “spatial normalization” to a common template to facilitate comparisons. Most fMRI studies utilize a standardized adult template;
using such a template may be problematical in pediatric studies, though, given that brain size and morphology change over the course of development. In typically developing children, this issue can be addressed by using a standardized pediatric template (12). However, in pediatric clinical populations in which distinct morphological differences exist, such as ADHD, the use of a template based only on typically developing children may create difficulties, leading to group activation differences that are due to better anatomical normalization in the typically developing group rather than actual differences in brain function. In these situations, creating a customized template based on the control and affected sample is preferable as it would allow equal representation of both groups in the template. An alternative or complementary approach, especially important in populations with noted reductions in brain volume, is covarying functional activation with local amounts of gray matter to determine whether differences in activation are due to functional abnormalities or differences in neuronal mass/registration error (13).
using such a template may be problematical in pediatric studies, though, given that brain size and morphology change over the course of development. In typically developing children, this issue can be addressed by using a standardized pediatric template (12). However, in pediatric clinical populations in which distinct morphological differences exist, such as ADHD, the use of a template based only on typically developing children may create difficulties, leading to group activation differences that are due to better anatomical normalization in the typically developing group rather than actual differences in brain function. In these situations, creating a customized template based on the control and affected sample is preferable as it would allow equal representation of both groups in the template. An alternative or complementary approach, especially important in populations with noted reductions in brain volume, is covarying functional activation with local amounts of gray matter to determine whether differences in activation are due to functional abnormalities or differences in neuronal mass/registration error (13).
Functional Magnetic Resonance Imaging Studies of Attention Deficit Hyperactivity Disorder
The biological substrates of ADHD are still uncertain, despite the fact that the disorder affects about 5% of school-aged children and frequently persists into adulthood (14). Understanding the neural basis of ADHD is critical to improving accurate diagnosis and helping to guide treatment. Recent studies have focused on three sets of neural findings in ADHD: abnormalities in response inhibition and resting state and neural effects of pharmacotherapy.
RESPONSE INHIBITION IN ATTENTION DEFICIT HYPERACTIVITY DISORDER
The clinical features in ADHD, including hyperactivity and impulsivity, and findings from neuropsychological studies (15, 16, 17) have contributed to leading hypotheses that ADHD is associated with impairments in executive control functions. There has been a particular emphasis on response inhibition (18), which refers to an inability to suppress behaviors that are inappropriate in a given context, and this has been the most examined domain in fMRI studies of ADHD. In the experimental setting, the most widely used tasks to assess response inhibition are the Go/No-go task and the Stop Signal task. In both of these tasks, a series of cued simple motor responses (“Go” trials requiring a button push) are accompanied by occasional “Stop” or “No-go” cues requiring suppression of this motor response. Impaired response inhibition, as measured by elevated commission error rates relative to typically developing children, is consistently observed in ADHD (15, 16, 17). Group differences in other behavioral measures have also been reported in ADHD. Specifically, children with ADHD show greater reaction times and reaction time variability during Go trials relative to typically developing children, suggesting that deficits in motor/behavioral control do not solely manifest as impaired response inhibition (17,19,20).
fMRI studies of response inhibition in “normal” adult populations have emphasized the involvement of circuits between the striatum and prefrontal regions, including the medial frontal wall, inferior frontal cortex and dorsolateral prefrontal cortex (21,22); however, localization of prefrontal activation is inconsistent across studies. These inconsistencies may be task dependent. One study examined healthy adults performing two versions of the Go/No-go task: a simple Go/No-go task with well-ingrained stimulus-response associations (green = Go, red = No-go) and a counting Go/No-go task in which the stimulus-response associations changed after each stimulus (green = Go, red = Go if preceded by
an even number of green stimuli, No-go if preceded by an odd number of green stimuli). The latter task requires constant manipulation of stimulus-response associations in working memory. Prefrontal activation in the simple task was localized to the rostral portion of the superior medial wall (pre-SMA), whereas greater activation in the counting task was present in right lateral prefrontal and inferior parietal regions as well as the pre-SMA, suggesting that these regions are recruited under conditions of increased working memory load (Fig. 9.2) (23). A similar systematic approach is warranted for examining differences in activation associated with response inhibition in both typically developing children and children with ADHD.
an even number of green stimuli, No-go if preceded by an odd number of green stimuli). The latter task requires constant manipulation of stimulus-response associations in working memory. Prefrontal activation in the simple task was localized to the rostral portion of the superior medial wall (pre-SMA), whereas greater activation in the counting task was present in right lateral prefrontal and inferior parietal regions as well as the pre-SMA, suggesting that these regions are recruited under conditions of increased working memory load (Fig. 9.2) (23). A similar systematic approach is warranted for examining differences in activation associated with response inhibition in both typically developing children and children with ADHD.
Current pediatric fMRI studies of response inhibition in ADHD are outlined in Table 9.1 (24, 25, 26, 27, 28, 29, 30, 31, 32, 33, 34, 35, 36). Most of the studies show differences in striatal and frontal regions; however, similar to what is seen in adults, frontal differences vary across studies and may be task dependent. Several of the tasks use novel stimuli, such as cartoon characters (27,33), objects (e.g., airplanes) (25,26,30), multiple letters (24,28,29,34), and arrows (31,32,35), which may have increased the need to maintain recently learned rules in working memory and necessitated recruitment of regions involved in working memory. In addition, tasks involving multiple stimuli tend to involve the anterior cingulate cortex, which has a well-established role in error detection.
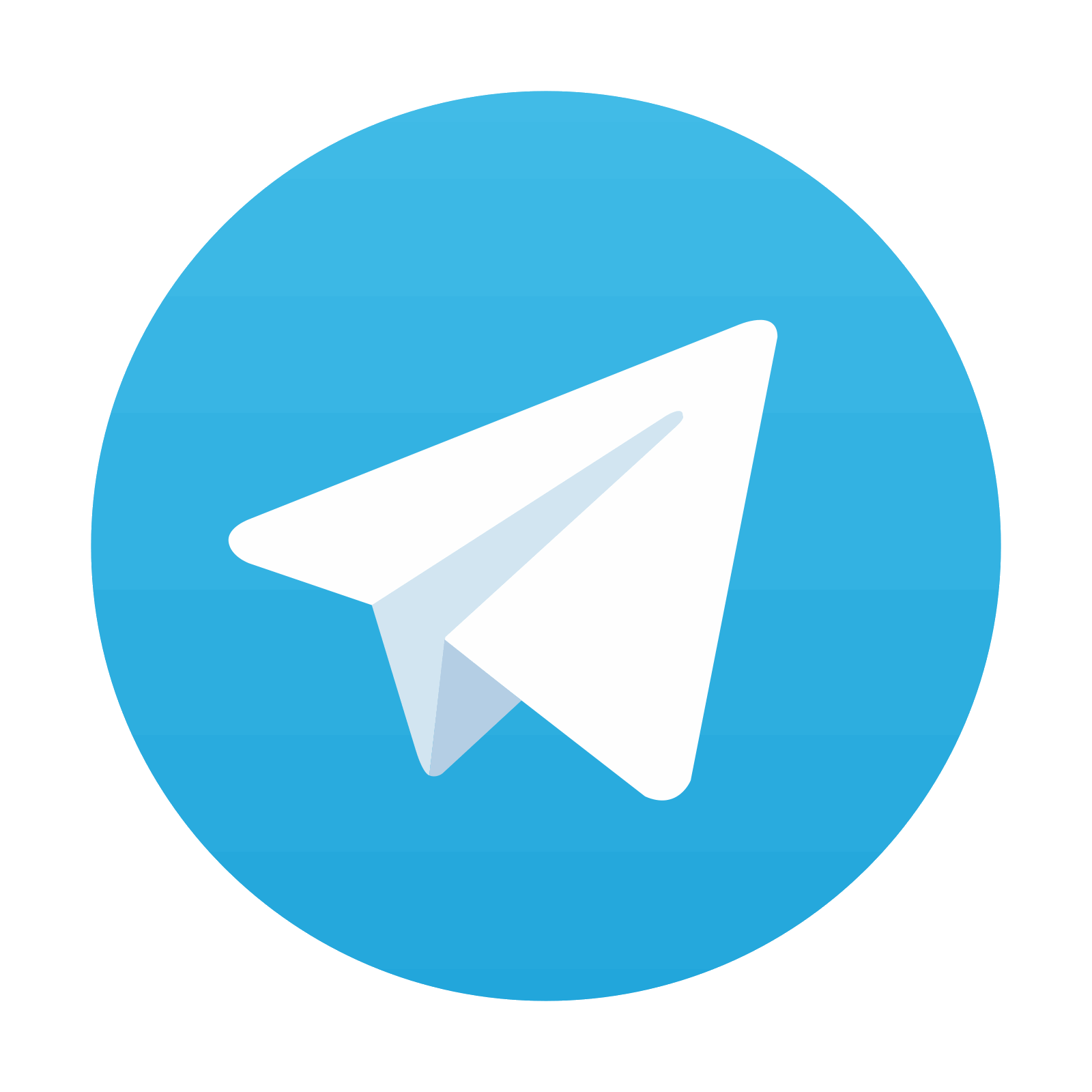
Stay updated, free articles. Join our Telegram channel
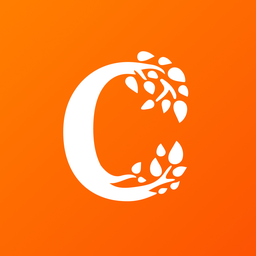
Full access? Get Clinical Tree
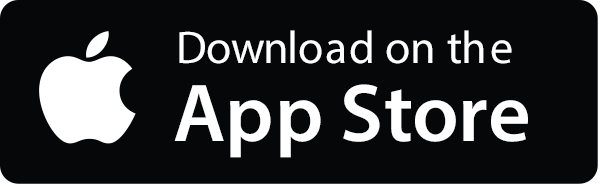
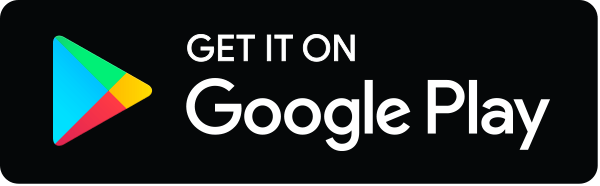