This chapter provides a brief introduction to fundamental aspects of electroencephalography (EEG), with a special emphasis on aspects of EEG that are particularly relevant to video-EEG monitoring (VEM). The sections below will summarize the biological basis of EEG, as well as its nomenclature, techniques for display, normal patterns, common artifacts, and relevant abnormalities. Most readers will already be familiar with common patterns found in the normal adult EEG, but several excellent references and atlases are available for more detailed discussions of these topics, as well as unusual variants and neonatal and pediatric EEG patterns.1–6 Although all components of routine EEG are applicable to VEM, several aspects of VEM introduce additional complexities in the acquisition, display, and accurate interpretation of the EEG. These aspects are discussed in more detail in subsequent chapters, with this chapter focusing on routine EEG interpretation for the beginning electroencephalographer.
Despite significant advancements in the design and manufacture of digital EEG and VEM systems, the active engagement of an experienced EEG technician remains a critical component for the reliable production and interpretation of EEG recordings. The introduction of digital media for both EEG and video has eliminated many routine maintenance tasks that historically fell under the supervision of EEG technicians, such as adjusting recording parameters and montages, ensuring the proper function of the ink writer, and accurately annotating the study with descriptions of behaviors or circumstances that may alter the EEG recording. Nevertheless, correct positioning and maintenance of electrodes; identifying, eliminating, or minimizing artifact in the modern acute care setting; and supervising the reliable and efficient storage of digital data have introduced new demands on the EEG technician (see Chapters 2 and 4).
Surface EEG represents a dynamic spatiotemporal summation of the extracellular current flow associated with excitatory and inhibitory postsynaptic potentials (EPSPs and IPSPs) as they reach their synaptic targets along the soma, axon, or dendritic tree of the pyramidal neuron.7 Thus, an EPSP along an apical dendrite produces positive current flowing into the cell (a current “sink”) and a corresponding extracellular field that is negatively charged. Passive current flowing out of the soma (a current “source”) maintains the electrical neutrality of the system but generates an extracellular electrical field, or dipole, in a radial orientation, with the negative pole directed toward the cortical surface (Figure 1-1). These extracellular dipoles are more prolonged and more spatially synchronized than action potentials mediated by fast sodium channels. Thus, the spatiotemporal summation of extracellular dipoles is the most likely biological source of cortical electrical activity recorded in the EEG. Evidence for a prominent role for the thalamus in synchronizing both normal and pathologic EEG activity dates to the mid-20th century, when repetitive stimulation of the thalamus was shown to entrain rhythmic activity across a wide area of the cerebral cortex8 and later demonstrated to produce a bilaterally synchronous 3 Hz spike and slow-wave epileptiform pattern.9
Figure 1-1.
Cortical generators of the electroencephalographic (EEG) signal. The schematic of a pyramidal neuron illustrates the neurophysiological basis of the surface EEG with an excitatory synapse along the superficial segment of an apical dendrite. Excitatory neurotransmitters produce an influx of sodium and calcium ions and an excitatory postsynaptic potential (EPSP). The summation of these EPSPs and inhibitory postsynaptic potentials (IPSPs) is the apparent generator of the EEG signal.

All EEG recordings consist of an array of output channels, with each channel displaying the algebraic difference between two input channels. Each input channel registers the electrical field at its connected electrode, and the output channel is, by definition, a voltage because it is represents the difference between two electrical potentials. The input channels are sometimes referred to as G1 and G2, referring to their historical identifiers as grids located within early vacuum tube amplifiers. G1 designates the first input channel within the pair, and G2 designates the second. As such, they indicate the input channel’s order in the differential amplification and not the channel’s electrode location. Surface electrodes are typically located and secured to the surface of the head in accordance with the international 10-20 or 10-10 system, and scalp electrodes are typically indentified by their position according to one of these two systems (Figure 1-2).
Recognition of the input channel’s position in the pair is important when considering the output channel’s polarity. By convention, a net positive output potential is displayed as a downward deflection in the output channel, and a net negative potential is displayed as an upward deflection in the output channel (Figure 1-3). As a result of this polarity convention, a downward (positive) deflection occurs when the electrical field measured at G1 is more positive than the field at G2, and an upward (negative) deflection is produced when the electrical field at G1 is more negative than the field at G2. Reversal in the position of the two input channels will therefore produce an opposite deflection of equal amplitude in the output channel; thus, the direction of deflection in an output channel does not indicate the polarity of an electrical field on the head’s surface. Confusion may occur during interpretation of the EEG because the terms negative and positive may be used to describe either the deflection in an output channel or the electrical field at a surface electrode, so it is important to specify whether a particular polarity (i.e., positive or negative) refers to the deflection of an EEG wave or the electrical field itself.
Figure 1-3.
Polarity convention. (A) The upper channel (Fp2–F8) of this pair of bipolar channels within a longitudinal chain demonstrates a downward deflection, indicating a net positive voltage; that is, the electrical field at G1 (Fp2) is greater than the field at G2 (F8). Conversely, the second channel (F8–T4) demonstrates an upward deflection, indicating a net negative voltage; i.e., the electrical field at G1 (F8) is less than the field at G2 (T4). When bipolar channels are displayed in this manner, transient surface negative electrical fields generate a negative phase reversal that is easily identified by the opposing deflections. (B) All three surface electrodes employed in A are now linked to the Cz electrode in a referential montage, and all three channels demonstrate upward deflections because the electrical field at the G1 electrodes (Fp2, F8, and T4) are more negative than the field at the referential G2 electrode (Cz). The wave demonstrating the highest amplitude (F8) reveals the location of maximal surface negativity.

The arrangement of output channels on an EEG display page is referred to as a montage, or in older literature, a recording “technique.” Many EEG montages are currently in use (Table 1-1); most commercial digital EEG systems contain several commonly used montages, and centers and practices may use these or montages of their own design. Regardless, there is no standard or universal montage. Prior to the development of computerized EEG, montages were established during the recording and could not be changed during subsequent interpretation of the EEG. Thus, there was limited opportunity to directly compare the appearance of a particular EEG pattern in different montages. This resulted in substantial debate over the advantages and disadvantages of different montages for revealing EEG abnormalities,10 as particular EEG patterns may appear quite different depending on the display montage, and different EEG montages are susceptible to different types of artifact. However, this problem has virtually disappeared with digital EEG, which allows shifting between different display montages during review. Thus, it is no longer necessary to choose a single best montage for a given EEG pattern. Indeed, the principal advantage of digital EEG is the ability to review a given pattern in varying forms as it is displayed in different montages.
Bipolar | Referential |
---|---|
|
|
Montages may be divided into two basic types: bipolar and common reference. Bipolar montages consist of output channels that include G1s and G2s as adjacent electrodes across the surface of the scalp. Most commonly, a particular input channel (i.e., an electrode) will be employed as G2 in a given output channel and G1 in the next output channel, producing a “chain” of channels that move through a line across the scalp. As an example, a commonly used montage includes four longitudinal lines, two parasagittal and two lateral (Figure 1-4A). This montage has been called the “double banana” because of its configuration as viewed diagrammatically from above. Other bipolar montages may include both longitudinal and transverse chains in a two-dimensional arrangement that has advantages for localization (Figure 1-4B–D). The principal advantage of a bipolar montage is that a focal negative or positive electrical potential is easily recognized because of the change in deflection in adjacent channels. The assignment of a particular input channel as G1 in a given output channel, and as G2 in the next output channel, generates this negative phase reversal that appears when output channel 1 registers a downward deflection, and output channel 2 registers an upward deflection. Similarly, a positive phase reversal appears when output channel 1 registers an upward deflection, and output channel 2 registers a downward deflection (Figure 1-3). A second advantage of bipolar montages is the fact that output channels reflect a more restricted electrical field across the scalp. Artifacts and cerebral fluctuations beyond the electrodes included in the chains have minimal impact on the overall appearance of the EEG.
Figure 1-4.
Selection of bipolar and referential montages. (A) A longitudinal bipolar (“double banana”) montage consists of bilateral temporal (red) and parasagittal (green) chains. (B) The combination of longitudinal and transverse bipolar chains includes bilateral temporal and parasagittal chains as in A in addition to a central chain (blue) that is particularly useful in indentifying vertex activity. (C) A circumferential “hatband” bipolar montage that includes both frontal (red) and occipital (green) chains is particularly useful in identifying abnormal transients over the frontal and occipital poles. This is complemented by three short transverse chains (blue). (D) This temporal bipolar montage includes bilateral temporal and parasagittal chains as in A, a frontal transverse chain, and a “halo” chain that begins at the vertex (black arrow), descends along the left centrotemporal region to the left anterior cheek electrode (T1), links left and right anterior cheek electrodes, and ascends the right centrotemporal region. This arrangement is particularly sensitive to anterior temporal lobe activity. (E) A Cz referential montage employs a referential electrode located at the vertex to compare contralateral homotopic regions. (F) An ipsilateral ear referential montage is useful for characterizing patterns with a broad field and/or a parasagittal location.

Common reference montages, more simply called referential montages, have input channel configurations in which the electrical field at various G1 “active” electrodes are compared with the G2 “referential” electrode, which is limited to one or two locations, such as the vertex (Cz) or ipsilateral ear (A1/A2) (Figure 1-4E, F). Alternatively, the “reference” may be a value automatically calculated by a computerized EEG system, such as the mathematical average of all recording electrodes. Historically, referential recordings were referred to as “unipolar,” and the reference electrode was sometimes referred to as a “neutral” or “indifferent” electrode, but these older terms are misleading because the reference electrode is not electrically neutral but will in fact register cerebrally generated electrical fields as well as physiological artifacts. The principal advantage of referential montages is most evident when the reference electrode is remote from the electrical field recorded by the active electrode. When this occurs, a referential montage provides a more accurate depiction of the amplitude and distribution of the electrical field at a number of active electrodes. Thus, bipolar and common reference montages complement one another because they differentially represent focal and diffuse electrical fields. Familiarity with the advantages and limitations of both bipolar and referential montages is a necessary skill for interpretation of EEG.
There are a number of additional parameters that will significantly alter the appearance of the surface EEG, including sensitivity (μV/mm), time scale (mm/s), and low- and high-frequency filters. Most commercial EEG and VEM systems include standard settings for these parameters, but their manipulation, in addition to shifting between montages, may help to minimize artifacts and identify subtle abnormalities in the EEG. It is important to note that, although most routine EEG and VEM studies employ a standard time scale of 30 mm/second for both acquisition and review, neonatal EEGs are reviewed with a compressed scale of 15 mm/second due to slower electrical conduction and rhythmic activity in the neonatal brain (see below), and neonatal EEGs are accompanied by additional channels to monitor respiratory patterns and muscle activity.
A common and easily recognizable EEG pattern in adults and older children consists of a sinusoidal rhythm, usually <50 mV in amplitude, that is present over the occipital, parietal, and/or posterior temporal regions during a state of relaxed wakefulness with eyes closed (Figure 1-5). This normal pattern is referred to as the posterior dominant (PDR) or alpha rhythm, the latter term dating back to Berger’s original description of the human EEG in 1929.11 The PDR will attenuate or “block” with eye opening or mental concentration, and may briefly appear with an increased frequency within the first 500 msec after eye closure, a phenomenon termed alpha “squeak.” The overall appearance of the PDR is stable in a healthy individual over time, although mild slowing of the PDR is common in elderly patients. By definition, a normal posterior dominant rhythm is within the alpha bandwidth of 8 to 13 Hz, and it typically is between 8.5 and 10.5 Hz (Table 1-2). Less commonly, rhythmic activity within the alpha range will be present over the right and/or left frontocentral head region. This normal variant is identified as a mu rhythm (Figure 1-6). The mu rhythm does not block with eye opening, but instead blocks with contralateral hand movement or thought of hand movement. As such, it is analogous to the alpha rhythm (PDR).
Figure 1-5.
Normal posterior dominant rhythm (PDR) or alpha rhythm. The 11 Hz posterior sinusoidal rhythm is “blocked” or attenuates with brief eye opening (solid arrow) and returns approximately 1 sec later with eye closure. Note that during the initial 500 msec following eye closure (open arrow), the PDR is faster, a phenomenon referred to as alpha “squeak.”

Frequency/Band | Normal Examples | State/Location/Features |
---|---|---|
Alpha (8–13 Hz) | PDR |
|
Mu rhythm |
| |
Beta (13–30 Hz) | Frontal beta |
|
Central beta |
| |
Sleep spindles (12–14 Hz) |
| |
Theta (4–8 Hz) | Generalized |
|
Posterior (7–8 Hz) |
| |
Ciganek rhythm (5–6 Hz) |
| |
RMTTD (5–7 Hz) |
| |
Delta (> 4 Hz) | Posterior slow waves of youth (2–3 Hz) |
|
Hyperventilation hypersynchrony |
| |
Hypnogogic hypersynchrony |
|
Electrical oscillations of the brain with frequencies >13 Hz constitute beta activity. The bony skull constitutes a high-frequency filter, such that normal beta activity is attenuated when recorded by scalp electrodes in subjects with an intact cranium; therefore, beta activity typically appears with a voltage <20 μV. Berger initially believed that beta activity was the manifestation of normal metabolic activity of cortical tissue,11 and early pioneers of EEG demonstrated that beta activity during the awake state develops its maximal amplitude over the frontal lobes.12 Indeed, intracranial studies have shown that faster oscillations in the 20 to 50 Hz range, referred to as gamma activity, predominate over the pre- and postcentral regions, and even faster high-frequency oscillations (HFOs) from 80 to 600 Hz have been recorded from depth electrodes within the human hippocampus.13
The appearance of beta activity in the normal EEG changes considerably as the subject transitions from wakefulness to sleep stages I and II and slow-wave sleep (Figures 1-5, 1-7, and 1-8). During the awake resting state, low-amplitude beta activity may be intermittently present over the frontal lobes, but recordings from particularly anxious or vigilant patients may contain prominent bifrontal or bilateral generalized beta activity. The appearance of high-amplitude beta activity that obscures background alpha or theta activity is frequently associated with benzodiazepines but can be seen with other agents as well. With the onset of stage I sleep, low-voltage (<20 μV) beta activity becomes more prominent over the perirolandic scalp electrodes (C3, Cz, and C4). Central beta may persist through stage II sleep but is typically absent during slow-wave sleep. During stage II and slow-wave sleep, well-organized packets of beta activity appear in the form of familiar sleep spindles over the vertex, with a typical frequency of 12 to 14 Hz, but spindles may appear as slow as 10 Hz and as fast as 16 Hz in some individuals.
Rhythmic activity within the theta range (4–8 Hz) is frequently present in the normal adult EEG during drowsiness or sleep. In infants and children, theta activity is the predominant component of the EEG background, with the PDR gradually increasing in frequency through early childhood from the fast theta range (6–8 Hz) into the alpha range. Individual waves within the theta bandwidth may intrude into the faster alpha rhythm in younger and older adults, and rhythmic theta activity is commonly seen in normal adults in three different locations. During the drowsy or stage I sleep state, attenuation and slowing of the PDR into the theta range is very common and typically results in a posterior theta rhythm of 7 to 8 Hz with a broad field (Figures 1-9 and 1-10). A Cigánek rhythm consisting of a 5 to 6 Hz oscillation may be present over the midline frontal or central regions during drowsiness (Figure 1-11). Lastly, rhythmic midtemporal theta of drowsiness refers to the appearance of rhythmic theta activity, usually 5 to 7 Hz, over the temporal lobe(s) during early stage I sleep (Figure 1-10). This is a pattern of historical controversy that was originally termed psychomotor variant due to its similarity to the electrographic appearance of temporal lobe (previously called psychomotor) seizures, but it is now considered a normal variant.
Delta activity (0.5–4.0 Hz) in the EEG of an awake adult is almost always abnormal. These slow waves were so named because of their early association with “disease, degeneration, and death.”14 There are two important exceptions: (1) Hypersynchronous delta is a normal response to hyperventilation in children and young adults; this pattern appears as high-amplitude 3 to 5 Hz activity during hyperventilation or in the immediate posthyperventilation period (Figure 1-12). (2) Posterior slow (i.e., 2–3 Hz) waves of youth with superimposed rhythmic alpha activity are common during childhood and may persist into early adulthood (Figure 1-13). During states of drowsiness, hypnagogic and hypnopompic hypersynchronous delta also can produce slowing in healthy young adults and children (Figure 1-14). Frequent or continuous polymorphic delta activity is a hallmark of slow-wave sleep in normal subjects (Figure 1-15), but it may be absent from many VEM studies due to altered sleep patterns in the acute care setting and/or frequent interruptions during the night. During the neonatal period, the normal EEG may consist of a discontinuous background when periods of mixed delta and theta activity are separated by periods of relative attenuation. This background evolves into a continuous pattern of delta and theta activity (Figure 1-16) that may include prominent sleep spindles by 2 months of age (Figure 1-17) and acquires a PDR pattern during wakefulness by 3 to 6 months of life.
Figure 1-13.
Posterior slow waves of youth present in a 13-year-old girl during the drowsy state. Note that these slow waves are most prominent in channels that include the occipital electrodes and appear as 2 to 3 Hz waves (arrows), often with superposition of the PDR. Beta activity and intermittent delta slowing are present over the vertex, and frontopolar channels reflect slow ocular movements of stage I sleep.

Figure 1-14.
A burst of hypnagogic hypersynchrony (arrow) during stage I sleep as indicated by the loss of PDR, the presence of central beta activity, and a vertex transient.
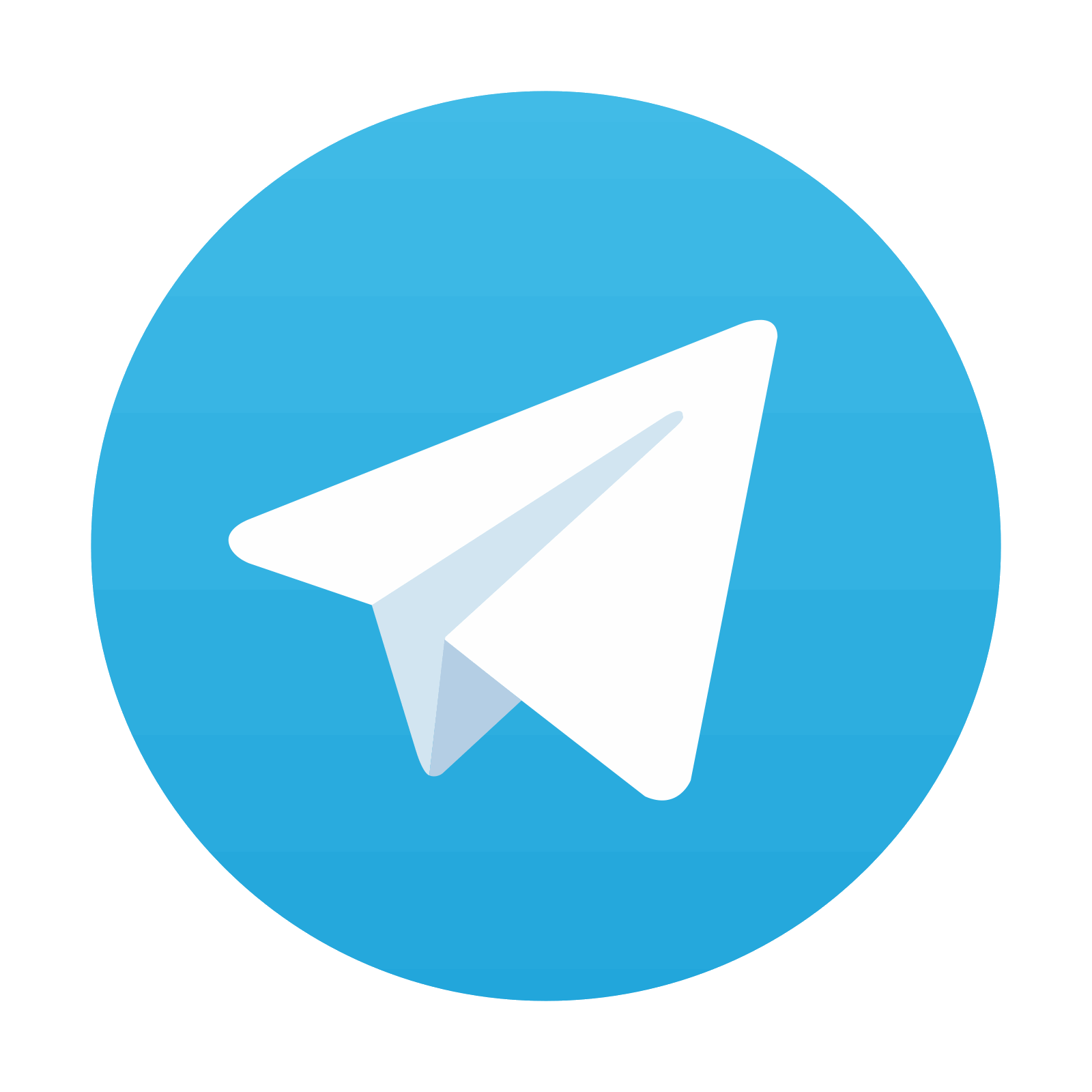
Stay updated, free articles. Join our Telegram channel
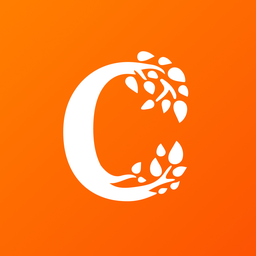
Full access? Get Clinical Tree
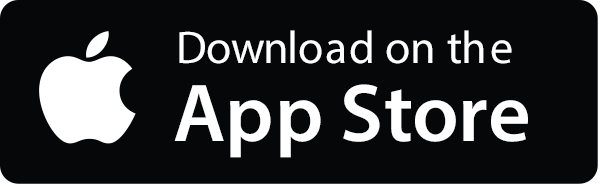
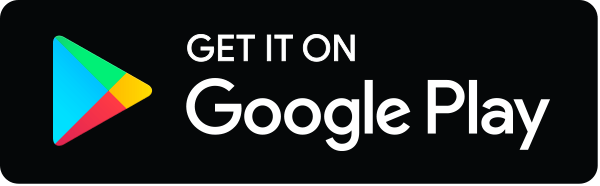
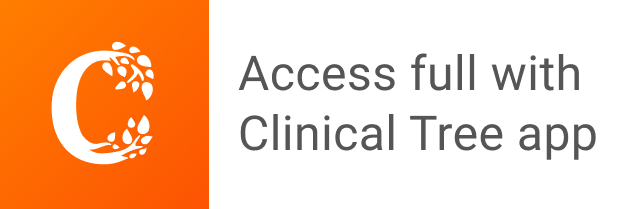