Fig. 10.1
The first electron microscopic images showing what the authors termed “abortive remyelination” – spontaneous myelin repair – at the edge of a multiple sclerosis plaque (Reproduced with permission of Oxford University Press; Perier and Gregoire [68])
Finally, moving away from cell biology and toward disease pathophysiology, although inflammatory demyelinating lesions are (still) commonly agreed to provide the pathophysiological substrate for relapses, it is increasingly considered that chronic disability in MS is more closely correlated with neuronal and axonal damage in the brain and spinal cord – and, no less relevantly, that neuroaxonal change outside individual lesions, of a far more diffuse nature may contribute significantly to progression [145–148]. It is therefore quite possible that repairing discrete lesions might not usefully affect chronic progressive disability.
To summarize the above in a rather extreme way, the conventional cell therapeutic approach in MS has proposed to add neural stem or myelinating cells to lesions that (a) already have stem cells, (b) already have oligodendrocyte precursors, (c) already have myelin, and (d) make no proven contribution to neurological functional impairment.
10.4 Novel Cell Therapy Approaches
An alternative summary of the foregoing would be that many very different studies over the last decade have yielded findings in multiple sclerosis that were in many ways surprising and counterintuitive, and so raised more (and deeper) questions than they answered. We still have an enormous amount to learn – more than was perhaps thought a decade ago – concerning the cell biology and the clinical and pathological physiology of MS. One obvious and very rational response to this might be to postpone any thought of clinical exploration of cell therapy in MS until we understand the disease better.
An alternative case can, however, be made. While securing a full understanding of disease processes before making efforts toward clinical translation is plainly ideal, it is hardly (if ever) realistic. There are few diseases whose mechanisms have been completely dissected – taking the example of MS itself, our comprehension of the immune disturbance is not remotely complete, and indeed neither do we have any real idea of what (biologically and immunologically) beta-interferon does in MS patients, but of course neither of these patches of partial ignorance prevented clinical trials of interferon in MS. What these changes in our perception of MS processes do mean, however, is that our intentions and designs in exploring cell therapy must evolve, and our demands of the “therapeutic” cell to be used in particular are different and more complex.
Just as immunotherapy studies clearly showed that stopping inflammation in patients with progressive disease did not have the hoped-for downstream effect of stopping progression (or by implication neurodegeneration), so it cannot be assumed that reversing demyelination – i.e., causing myelin repair – will stop axonal degeneration. Given the many areas of uncertainty outlined above, a “perfect” cell in any putative reparative therapy in MS needs to have distinct and separate beneficial effects on as many of the known adverse processes in this disease as possible. Ideally, such a cell would modulate immune processes both systemically and inside the CNS, and so dampen inflammation in the brain and spinal cord. Additionally, and through distinct mechanisms, our ideal cell would have remyelinating activity; and it would have separate axon- and neuroprotective and/or reparative effects. What is more, we would need to demand of our cell that it be capable of exhibiting these effects both within lesions and in white and grey matter outside and between lesions.
Whether or not this is a realizable “shopping list” of desired properties, it is arguably, now, a more realistic place to start in considering repair therapies in MS than the classical “injecting myelinating cells into lesions” paradigm. And although it seems highly unlikely that any one single cell type, stem cell or otherwise, could fully and efficiently meet every one of these demands, rapid recent advances in our knowledge of some reparative cells indicates that the challenges may not be quite so impossible. This perhaps applies in particular to bone marrow-derived mesenchymal stem cells.
Bone marrow (BM) derived stem cells have been considered attractive candidates for cell therapies in a wide range of diseases, not least because the normal function of some BM cell subpopulations, mesenchymal stem cells (MSCs) having perhaps been most carefully studied, is spontaneous tissue repair [149, 150]; and furthermore that they execute this function through multiple and very varied reparative mechanisms [150, 151]. While the true “stem-ness” of mesenchymal stem cells remains open to question, it may well be the case paradoxically that amongst their properties, transdifferentiation, at least into oligodendrocytes, is the least important in the context of chronic progressive MS. As outlined above, cell therapy in MS may well no longer have as its core aim the replacing myelinating cells to regenerate myelin, and other properties of these cells may be at least as important.
First, they have long been known to exhibit pronounced immune-modulating and immune suppressive properties, with both local and systemic effects of significant possible relevance to MS [152–155]. Second, both whole, unseparated, bone marrow cells and isolated MSCs have been shown to promote myelin repair following experimental demyelination – and significantly, such pro-remyelinating activity is seen not just in experimental inflammatory demyelination (where the beneficial effects might well be secondary to their immune suppressive functions), but in toxic or chemical, nonimmune experimental demyelination [156, 157]. The mechanisms of this effect remain uncertain. Initially it was thought that transdifferentiation into myelinating cells was responsible [102–105, 158, 159], but as mentioned above, transdifferentiation of MSCs into functional, myelin-elaborating oligodendrocytes is seriously questioned [160, 161] (though not entirely settled [108, 109]). Alternative, indirect pro-remyelination effects may be more likely – stimulating the proliferation of endogenous neural stem cells [162] and directing their differentiation along oligodendrocyte pathways [163–165], for example.
Other properties could also be important. MSCs can reduce gliotic scar formation – and gliosis is considered a major barrier to spontaneous repair in MS [165–167]. MSCs have powerful neuroprotective properties [168–172], again independent of their anti-inflammatory activity, although protection against oxidative stress applies to both immune and nonimmune tissue damage [173, 174]. Also, and again as a distinct property, fusion of MSCs with some cell types (perhaps, particularly, cerebellar Purkinje cells) may represent a further mechanism by which MSCs may offer protection (or “rescue” damaged cells) [175, 176].
With the single though notable exception of these cells’ systemic effects on immunity, all of these listed potentially valuable therapeutic capacities of MSCs could have no value in multiple sclerosis unless the cells were able to gain close proximity to areas of tissue damage, and this is where a final crucial property of MSCs becomes relevant. Circulating MSCs have long been known to “home” specifically to areas of tissue damage or inflammation [149, 177, 178]. This applies not only to endogenous cells released from the marrow in health and disease, but also to exogenous cells, delivered intravenously, which successfully infiltrate the brain and spinal cord, and are stimulated to migrate toward those cytokines known to be expressed in MS lesions [179, 180].
Two further points should be emphasized. All these studies of potential therapeutic mechanisms of action center on bone marrow mesenchymal cells. However, it is firstly the case that other tissues – indeed, probably all adult tissues – also harbor MSCs [181], and such tissues may also serve as a source of therapeutic cells [182]. No less importantly, other subpopulations of bone marrow cells also have the capacity to contribute to and promote tissue repair, also by utilizing a wide variety of effector mechanisms, including hematopoetic stem cells (HSCs) [104, 183–187], Stro-1 positive cells [188–192], CD133+ve cells [193–195], MAPCs, and others [108, 109, 196]. Many of these subtypes have as yet been subject to far less intensive experimental exploration than MSCs, but this alone is not evidence that they will prove any less interesting or potentially valuable. MSCs themselves are not likely to be a single, homogenous cell type: subvarieties are probable [150, 197].
Remarkably, there is powerful in vivo evidence in humans of the capacities of migration and successful functional differentiation of bone marrow-derived cells. Various tissues from bone marrow transplant recipients who received cells from sex-mismatched donors (as treatments for leukemias and related conditions) have been examined in carefully executed and highly informative autopsy studies for the presence of donor-derived (i.e., “wrong” sex) cells. Such studies reveal that intravenously delivered bone marrow cells do indeed infiltrate throughout the human CNS, apparently remain in place for years if not decades [198, 199], and are identifiable as highly differentiated neuronal and glial phenotypes fully integrated into the host brain. (Such studies do not, however, shed information on which particular bone marrow cell type(s) yielded these CNS-resident cells.)
The hazards of using embryo-derived cells in patients were mentioned above, but it would be wrong to assume that adult stem cells, simply though not being embryonic, were necessarily wholly free from hazard [200]. Safety must remain paramount: multiple sclerosis is a chronic but nonfatal disease, making therapies with significant and predictable risks far less tolerable than in, say, cancer treatments or those with serious cardiac disease. The repeated cell cycling and expansion required to prepare purified, selected MSCs (or other subpopulations) may induce unwelcome genetic instability [201–203] – and can also compromise differentiation and repair capacities [204–208]. However, malignant, donor-derived tumors have not been reported in patients receiving MSCs, including the now several hundred treated in trials for various cardiac diseases [209]. Nonetheless, a recent report does indicate that MSCs directly injected intra-ventricularly can form non-proliferating cellular mass lesions; [210, 211] other studies have not found the same, though one reported axon-damaging effects (and no beneficial consequences) of direct intra-parenchymal injection of MSCs in rodents [212].
The use of nonselected, non-expanded bone marrow cells would eliminate the potentially unwanted effects of cell culture and expansion (and, if there were to be comparable benefit, would be far easier and cheaper to adopt and apply widely and in relatively nonspecialist units, with no requirement for a sterile GMP cell growth or selection facility). This approach also bypasses the question of choosing one specific BM stem cell subtype to study clinically – cell selection obviously excludes the majority of cell types, but as mentioned above, there is no good reason to exclude any specific BM stem cell subpopulation. Using unselected BM cells is an increasingly common approach, successfully explored not just in experimental models of stroke [213] and spinal cord injury [214], but also clinically, in patients with myocardial infarction [215, 216], liver disease [217] and peripheral vascular disease [218], and more recently stroke [219]. Indeed in some circumstances, unfiltered cells have been directly compared with purified MSCs and shown to better reparative potential [220, 221]. Certainly, in MS, this approach would mimic the relatively unselected mononuclear cell population that proved beneficial in the original therapeutic studies in rodent demyelinating models [156, 222]. For these and other reasons, nonselected mononuclear cells were used in the Bristol safety and feasibility study of bone marrow cell therapy in MS [223], though intravenously and intrathecally delivered mesenchymal stem cells were used in a more recently published early phase trial [224]. Both studies confirmed safety, but no less relevantly indicated that the clinical exploration of reparative cell therapy in patients with progressive multiple sclerosis has at last commenced.
References
1.
Scalfari A, et al. The natural history of multiple sclerosis: a geographically based study 10: relapses and long-term disability. Brain. 2010;133:1914–29.PubMed
2.
Confavreux C, Vukusic S, Moreau T, Adeleine P. Relapses and progression of disability in multiple sclerosis. N Engl J Med. 2000;343:1430–8.PubMed
3.
Coles AJ, et al. Monoclonal antibody treatment exposes three mechanisms underlying the clinical course of multiple sclerosis. Ann Neurol. 1999;46:296–304.PubMed
4.
Campbell GR, et al. Mitochondrial DNA deletions and neurodegeneration in multiple sclerosis. Ann Neurol. 2011;69:481–92.PubMed
5.
Lassmann H. Mechanisms of neurodegeneration shared between multiple sclerosis and Alzheimer’s disease. J Neural Transm. 2011;118:747–52.PubMed
6.
Witherick J, Wilkins A, Scolding N, Kemp K. Mechanisms of oxidative damage in multiple sclerosis and a cell therapy approach to treatment. Autoimmune Dis. 2010;2011:164608.PubMed
7.
Rojas JI, Romano M, Ciapponi A, Patrucco L, Cristiano E. Interferon beta for primary progressive multiple sclerosis. Cochrane Database Syst Rev. 2010:CD006643.
8.
Hawker K, et al. Rituximab in patients with primary progressive multiple sclerosis: results of a randomized double-blind placebo-controlled multicenter trial. Ann Neurol. 2009;66:460–71.PubMed
9.
Wolinsky JS, et al. Glatiramer acetate in primary progressive multiple sclerosis: results of a multinational, multicenter, double-blind, placebo-controlled trial. Ann Neurol. 2007;61:14–24.PubMed
11.
Rodriguez M. A function of myelin is to protect axons from subsequent injury: implications for deficits in multiple sclerosis. Brain. 2003;126:751–2.PubMed
12.
Bitsch A, Schuchardt J, Bunkowski S, Kuhlmann T, Bruck W. Acute axonal injury in multiple sclerosis. Correlation with demyelination and inflammation. Brain. 2000;123:1174–83.PubMed
13.
Kornek B, et al. Multiple sclerosis and chronic autoimmune encephalomyelitis: a comparative quantitative study of axonal injury in active, inactive, and remyelinated lesions. Am J Pathol. 2000;157:267–76.PubMed
14.
Lipton SA. Blockade of electrical-activity promotes the death of mammalian retinal ganglion-cells in culture. Proc Natl Acad Sci USA. 1986;83:9774–8.PubMed
15.
Raine CS, Cross AH. Axonal dystrophy as a consequence of long-term demyelination. Lab Invest. 1989;60:714–25.PubMed
16.
Griffiths I, et al. Axonal swellings and degeneration in mice lacking the major proteolipid of myelin. Science. 1998;280:1610–3.PubMed
17.
MeyerFranke A, Kaplan MR, Pfrieger FW, Barres BA. Characterization of the signaling interactions that promote the survival and growth of developing retinal ganglion cells in culture. Neuron. 1995;15:805–19.
18.
Wilkins A, Majed H, Layfield R, Compston A, Chandran S. Oligodendrocytes promote neuronal survival and axonal length by distinct intracellular mechanisms: a novel role for oligodendrocyte-derived glial cell line-derived neurotrophic factor. J Neurosci. 2003;23:4967–74.PubMed
19.
Lappe-Siefke C, et al. Disruption of Cnp1 uncouples oligodendroglial functions in axonal support and myelination. Nat Genet. 2003;33:366–74.PubMed
20.
Edgar JM, et al. Oligodendroglial modulation of fast axonal transport in a mouse model of hereditary spastic paraplegia. J Cell Biol. 2004;166:121–31.PubMed
21.
Irvine KA, Blakemore WF. Remyelination protects axons from demyelination-associated axon degeneration. Brain. 2008;131:1464–77.PubMed
22.
Gill SS, et al. Direct brain infusion of glial cell line-derived neurotrophic factor in Parkinson disease. Nat Med. 2003;9:589–95.PubMed
23.
Love S, et al. Glial cell line-derived neurotrophic factor induces neuronal sprouting in human brain. Nat Med. 2005;11:703–4.PubMed
24.
Lang AE, et al. Randomized controlled trial of intraputamenal glial cell line-derived neurotrophic factor infusion in Parkinson disease. Ann Neurol. 2006;59:459–66.PubMed
25.
Hutchinson M, Gurney S, Newson R. GDNF in Parkinson disease: an object lesson in the tyranny of type II. J Neurosci Methods. 2007;163:190–2.PubMed
26.
Patel NK, Gill SS. GDNF delivery for Parkinson’s disease. Acta Neurochir Suppl. 2007;97:135–54.PubMed
27.
Du Y, Dreyfus CF. Oligodendrocytes as providers of growth factors. J Neurosci Res. 2002;68:647–54.PubMed
28.
Wilkins A, Chandran S, Compston A. A role for oligodendrocyte-derived IGF-1 in trophic support of cortical neurons. Glia. 2001;36:48–57.PubMed
29.
Wilkins A, Scolding N. Protecting axons in multiple sclerosis. Mult Scler. 2008;14:1013–25.PubMed
30.
Frank JA, et al. A pilot study of recombinant insulin-like growth factor-1 in seven multiple sderosis patients. Mult Scler. 2002;8:24–9.PubMed
31.
Skaper SD. Peptide mimetics of neurotrophins and their receptors. Curr Pharm Des. 2011;17(25):2704–18.PubMed
32.
Dawbarn D, Allen SJ. Neurotrophins and neurodegeneration. Neuropathol Appl Neurobiol. 2003;29:211–30.PubMed
33.
Gilgun-Sherki Y, Panet H, Melamed E, Offen D. Riluzole suppresses experimental autoimmune encephalomyelitis: implications for the treatment of multiple sclerosis. Brain Res. 2003;989:196–204.PubMed
34.
Gonsette RE. Oxidative stress and excitotoxicity: a therapeutic issue in multiple sclerosis? Mult Scler. 2008;14:22–34.PubMed
35.
Loria F, et al. An endocannabinoid tone limits excitotoxicity in vitro and in a model of multiple sclerosis. Neurobiol Dis. 2010;37:166–76.PubMed
36.
Jackson SJ, Baker D, Cuzner ML, Diemel LT. Cannabinoid-mediated neuroprotection following interferon-gamma treatment in a three-dimensional mouse brain aggregate cell culture. Eur J Neurosci. 2004;20:2267–75.PubMed
37.
Jackson SJ, Diemel LT, Pryce G, Baker D. Cannabinoids and neuroprotection in CNS inflammatory disease. J Neurol Sci. 2005;233:21–5.PubMed
38.
Pryce G, et al. Cannabinoids inhibit neurodegeneration in models of multiple sclerosis. Brain. 2003;126:2191–202.PubMed
39.
Zajicek J, et al. Cannabinoids for treatment of spasticity and other symptoms related to multiple sclerosis (CAMS study): multicentre randomised placebo-controlled trial. Lancet. 2003;362:1517–26.PubMed
40.
Waxman SG. Mechanisms of disease: sodium channels and neuroprotection in multiple sclerosis-current status. Nat Clin Pract Neurol. 2008;4:159–69.PubMed
41.
Bechtold DA, Kapoor R, Smith KJ. Axonal protection using flecainide in experimental autoimmune encephalomyelitis. Ann Neurol. 2004;55:607–16.PubMed
42.
Black JA, Liu S, Hains BC, Saab CY, Waxman SG. Long-term protection of central axons with phenytoin in monophasic and chronic-relapsing EAE. Brain. 2006;129:3196–208.PubMed
43.
Kapoor R, Davies M, Blaker PA, Hall SM, Smith KJ. Blockers of sodium and calcium entry protect axons from nitric oxide-mediated degeneration. Ann Neurol. 2003;53:174–80.PubMed
44.
Kapoor R, et al. Lamotrigine for neuroprotection in secondary progressive multiple sclerosis: a randomised, double-blind, placebo-controlled, parallel-group trial. Lancet Neurol. 2010;9:681–8.PubMed
45.
Plane JM, Shen Y, Pleasure DE, Deng W. Prospects for minocycline neuroprotection. Arch Neurol. 2010;67:1442–8.PubMed
47.
Wilkins A, Nikodemova M, Compston A, Duncan I. Minocycline attenuates nitric oxide-mediated neuronal and axonal destruction in vitro. Neuron Glia Biol. 2004;1:297–305.PubMed
48.
Metz LM, et al. Minocycline reduces gadolinium-enhancing magnetic resonance imaging lesions in multiple sclerosis. Ann Neurol. 2004;55:756.PubMed
49.
Metz LM, et al. Glatiramer acetate in combination with minocycline in patients with relapsing – remitting multiple sclerosis: results of a Canadian, multicenter, double-blind, placebo-controlled trial. Mult Scler. 2009;15:1183–94.PubMed
50.
McPherson RJ, Juul SE. Recent trends in erythropoietin-mediated neuroprotection. Int J Dev Neurosci. 2008;26:103–11.PubMed
51.
Sattler MB, et al. Neuroprotective effects and intracellular signaling pathways of erythropoietin in a rat model of multiple sclerosis. Cell Death Differ. 2004;11 Suppl 2:S181–92; S181–92.PubMed
52.
Diem R, et al. Combined therapy with methylprednisolone and erythropoietin in a model of multiple sclerosis. Brain. 2005;128:375–85.PubMed
53.
Ehrenreich H, et al. Exploring recombinant human erythropoietin in chronic progressive multiple sclerosis. Brain. 2007;130(Pt 10):2577–88.PubMed
54.
Frank T, et al. Both systemic and local application of granulocyte-colony stimulating factor (G-CSF) is neuroprotective after retinal ganglion cell axotomy. BMC Neurosci. 2009;10:49.PubMed
55.
Xiao BG, Lu CZ, Link H. Cell biology and clinical promise of G-CSF: immunomodulation and neuroprotection. J Cell Mol Med. 2007;11:1272–90.PubMed
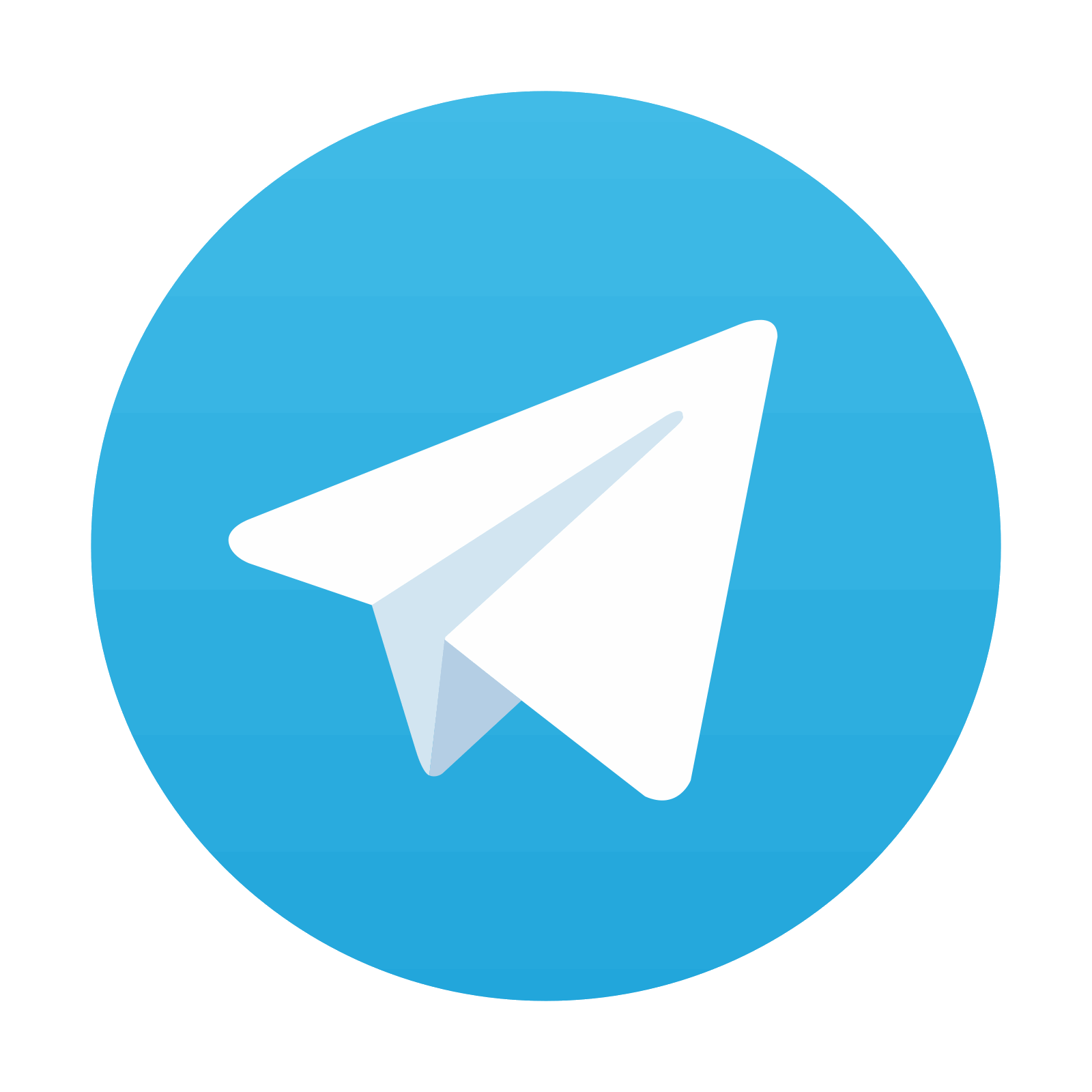
Stay updated, free articles. Join our Telegram channel
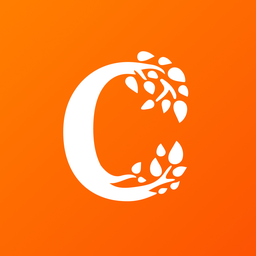
Full access? Get Clinical Tree
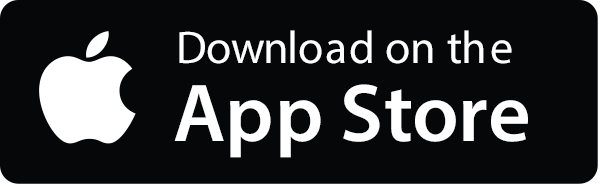
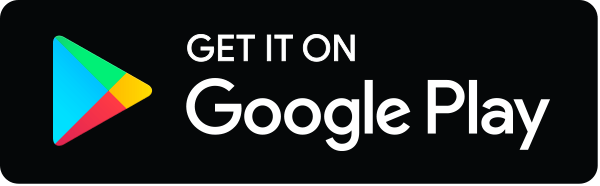