Serotype
Natural host/origin
Optimal organ/tissue/cell type transduced
CNS area transduced
Receptor
Coreceptor
Blood–brain barrier penetration
Disease targets
AAV1
Monkey
Unknown
Lipoprotein lipase deficiency [112]
AAV2
Human
HSPG [64]
AAV3
Human
Liver cancer cells [110]
HSPG [124]
AAV4
African green monkey
2,3O-linked sialic acid [127]
Unknown
AAV5
Human
2,3 N-sialic acid [128]
PDGFR [129]
Rheumatoid arthritis [130]
AAV6
Human
Muscle, liver, spinalcord [110]
Spinal cord [110]
2,3 N/2,6 N-sialic acid [111]
EGFR [131]
AAV7
Rhesus monkey
N-sialic acid [110]
PDGFR [110]
AAV8
Rhesus monkey
Unknown
LamR [116]
Hemophilia B [46]
AAV9
Cynomolgus monkey
N-galactose [135]
LamR [116]
AAV10
Cynomolgus monkey
Unknown
Unknown
AAV11
Cynomolgus monkey
Spleen, lymph node [136]
Unknown
Unknown
AAV12
African green monkey
Nasal epithelia [138]
Unknown
Unknown
AAV2.5
AAV2 with 5 amino acid mutations from AAV1
Muscle [49]
Duchenne muscular dystrophy [49]
AAV hybrid serotypes have capsid protein modifications that increase the specificity, efficiency, and efficacy of viral infection (for review, see ref. 35), which are essential for targeting the tissue and cell type of interest and minimizing dose. One variety of hybrid serotype is obtained through transcapsidation, or packaging the genome containing ITRs (cis-acting) from one serotype into the capsid of a different serotype (trans-acting virion shell). The tropism, or host cell specificity, is determined by the three cap genes; tropism of an AAV vector during transduction can thus be changed by exchanging the rep/cap gene source. Most vectors that were developed prior to the development of hybrid vectors have been AAV2, and these are most used as ITRs for cross-packaging in capsids of another serotype. Transcapsidation of the AAV2 genome allows the genome from this well-characterized serotype to target cell types that don’t express receptors utilized by AAV2. AAV2 is present in ~80–90 % of the population; AAV2-neutralizing antibodies were found in humans in early AAV studies [36] and are now known to be more prevalent than AAV1-neutralizing antibodies in human [37]. Thus, since tropism is determined by the cap genes, rAAV has been developed with AAV2 ITRs and rep and cap genes of alternative serotypes, thereby avoiding actions of AAV2-neutralizing antibodies. AAV2 ITRs are used because AAV2 is the most understood serotype, in terms of host cell response as well as biochemical and genetic properties; transcapsidation with alternate serotype capsids has expanded the utility of this prototypical vector.
By packaging the capsids of different serotypes with AAV2 ITRs, the transduction efficiencies of naturally occurring AAV serotype vectors in different tissues and cell types have been compared, providing a vast amount of information on specific serotypes in the literature. Keeping in mind inter-study variation in AAV promoters, transgenes, titers, and doses, comparative efficiency of transduction in major tissues has been established (see Table 1 for summary) [30, 38]. AAV2 transduces a wide range of cell types and tissues with moderate efficiency (the liver, muscle, lung, CNS). AAV9 has a similar profile to AAV2 but shows more efficient transduction [39]. In skeletal muscle, AAV1 and AAV7 show rapid onset and high transduction levels [39]. AAV6, because it differs from AAV1 by only six amino acids, also transduces skeletal muscle well.
In the nervous system, AAV1, 2, 5, 8, and 9 have been the most widely studied serotypes, with AAV1 and 5 conferring a superior transduction efficiency than AAV2, and AAV8 and 9 conferring further superior transduction over AAV1 and 5 in the rodent CNS [30, 32, 34, 40, 41]. In comparison to AAV2, most serotypes show greater CNS transduction in terms of total transduced brain volume and total number of transduced cells, but also in some cases, higher level of gene expression per cell [32, 40]. Burger et al. assess CNS transduction of AAV1, 2, and 5 capsids flanked with AAV2 ITRs injected into different regions of the rat brain [32]. While all constructs primarily transduced neurons, AAV1 and AAV5 capsids show higher transduction efficiency than AAV2 in all CNS regions, including the hippocampus, striatum, globus pallidus, substantia nigra, and spinal cord. In the hippocampus, AAV1 and 5 capsids mostly transduce pyramidal neurons in CA1-CA3 regions, while AAV2 mostly transduces neurons in the hilar region of the dentate gyrus. AAV8 shows superior transduction to AAV1, 2, and 5 in the hippocampus of the rat brain, although one study shows that an efficient gene transfer of AAV8 causes neurotoxic green fluorescent protein (GFP) expression levels [40]. In contrast to rodent findings, a study in adult cynomolgus monkeys shows superior transduction by AAV1 and AAV5 compared to AAV8 in the striatum [42]. Klein et al. showed that AAV9 conferred more efficient gene transfer in the brain than AAV2 or AAV8 in rat tauopathy models [34]. For AD studies, researchers may be interested in injecting into many regions but perhaps specifically the hippocampal region, where early degeneration occurs. Thus, for targeting the hippocampus in rodent studies, AAV8 [40] and AAV9 [34] may be superior.
AAV9 has recently been studied for its unique ability to cross the blood–brain barrier (BBB), allowing administration in the periphery [41, 43–45]. In adult mice, AAV9 administered peripherally shows transduction of mainly neurons in the hippocampus and striatum, but mainly astrocytes in the cortex [41]; an opposing study reported robust transduction of AAV9 to astrocytes throughout the CNS with limited neuronal transduction [43]. To highlight the need for caution in extrapolating transduction efficiencies between species, AAV9 shows reduced transduction efficiency and a switch from primarily neuronal to primarily glial expression in nonhuman primates as compared to rodents, which could be attributed to differential low levels of preexisting AAV-neutralizing antibodies in the primates [46]. However, another study reports neuron-specific infection in the macaque and marmoset brain by AAV8-EGFP and AAV9-EGFP [47]. Although AAV9 is the only naturally occurring serotype known to show BBB permeability, numerous chimeric and directed evolution vectors have been constructed to cross the BBB, allowing peripheral administration of a CNS-targeted therapeutic gene [48]. Due to their increased specificity and transduction efficacy, pseudotyped vectors are now the primary choice for rAAV clinical trials. However, despite the focus on comparing serotypes, some researchers posit that serotype differences have a minimal overall effect on transduction efficacy [12, 34].
In addition to hybrid serotypes involving transcapsidation, rationally designed AAV capsids have driven the customization of AAV vectors to improve efficacy for clinical use, which include chimeric and mosaic capids and capsid mutants. For example, a capsid termed AAV2.5 was developed using AAV2 with five amino acid mutations from AAV1, in order to harness the muscle-transducing abilities of AAV1 with the receptor binding properties of AAV2 and to decrease antigenic cross-reactivity against both parental serotypes. AAV2.5 was subsequently used in a phase I clinical trial for Duchenne muscular dystrophy [49]. Additional AAV capsids have been designed to achieve unique tropisms and to improve transduction efficiency (for review, see ref. 28).
Promoters and enhancers are frequent components of rAAV vectors that can be used to boost viral efficacy and specificity of gene expression. rAAV constructed for use in neurological disease accordingly contain CNS cell type-specific promoters, including platelet-derived growth factor (PDGF) [50], neuron-specific enolase (NSE) promoter, chicken β-actin promoter (CBA), and the cytomegalovirus (CMV) promoter. The CMV promoter confers high neuron-selective transgene expression [23, 51], but some studies observed different durations of expression of vectors with this promoter depending on the brain region, implying region-specific CMV promoter suppression [24, 51]. CMV hybrid promoters, such as a CMV/CBA promoter developed by Niwa et al. [52], can prevent this attenuation of CMV promoter activity. CMV/CBA hybrid vectors were additionally found to transduce more cells than NSE vectors in rat hippocampus [53]. Alternatively, CMV promoter may be used for long-term expression after experimental confirmation that the promoter supports long-term expression in their brain area of interest. Gray et al. reported that CBA promoters show ubiquitous and high neural expression, but low expression in motor neurons [51]. This was bypassed by development of an 800-bp hybrid CBA promoter that shows high expression in motor neurons and all cell types transduced by native CBA and CMV promoters [51].
Some studies may aim for glial expression of transgene, in which case glial-specific promoters can be used. This may be useful for studying the involvement of glial cells in AD as well as for many neurodegenerative autoimmune diseases involving demyelination such as multiple sclerosis, Charcot–Marie–Tooth disease, and various leukodystrophies. The glial fibrillary acidic protein (GFAP) promoter has successfully been used for infection of astrocytes, while the myelin basic protein (MBP) promoter can be used for oligodendrocyte infection [54–56].
The vector also contains the woodchuck hepatitis virus posttranscriptional regulatory element (WPRE), a commonly used 3′UTR enhancer utilized to increase viral titer and transgene expression [57]. Klein et al. reported an 11-fold increase in transgene expression levels with incorporation of the WPRE into a CBA promoter vector in rat hippocampus, as measured by cell number and Western blot [53].
Due to the small size of the AAV genome, other viral vectors typically have a greater capacity with which to insert transgenes, but this has mostly been overcome by vector constructs specially engineered to carry large transgenes. For instance, split vectors have been made in which the transgene cassette is split into two constructs, which have a slight sequence overlap with each other so that recombination after vector nuclear entry leads to the intact transgene product being expressed [58]. Using this trans-splicing method, therapeutic genes up to 9 kb have been delivered in the retina, lung, and muscle, although these vectors are less efficient than normal rAAV vectors [59]. Alternatively, Gray et al. developed a short neuronal promoter that can package larger transgenes into AAV vectors because of the relatively reduced size of the promoter—a 229-bp fragment of the mouse methyl-CpG-binding protein-2 (MeCP2) [51].
1.3 Advancements in rAAV Production
As a complement to innovations in vector design, improvements in methods for rAAV manufacturing have advanced AAV gene therapy for human use. These include the engineering of plasmids carrying adenovirus helper functions without the complete adenovirus genome, stable “producer” cell lines providing helper functions, and purification methods resulting in large rAAV yields appropriate for clinical use.
Recombinant AAV was historically produced after gene construction by using wild-type adenovirus as a helper virus. This method involves transfecting cell lines that stably harbor AAV rep/cap genes with wild-type adenovirus and the AAV vector DNA. Although this can be scaled up to produce vectors with high titers, it is difficult to avoid adenovirus contamination in this method even with the use of repeated cesium chloride (CsCl) gradients. This has been overcome by the development of the “mini-Ad genome,” plasmids carrying only the adenovirus helper genes (which include the E1a, E1b, E2a, E4, and VA RNA genes). This adenovirus-free method involves transient transfection of all elements required for AAV production into host cells (such as HEK293 cells): an rAAV ITR-containing plasmid carrying the gene of interest, a plasmid that carries AAV rep and cap genes, and a helper plasmid with only the adenovirus helper genes. This triple transfection “helper-free” method was first used with the mini-Ad genome plasmid PXX6 [60], and since then similar plasmids have been developed, such as pFD13, which has an 8-kb deletion in the adenovirus E2B region and most of the late genes [37]. The mini-Ad genome preceded the development of pDG, a plasmid with AAV2 packaging functions and adenovirus helper functions (AAV2 rep and cap genes and VA, E2A, and E4 genes of adenovirus 5), allowing for transfection of only two plasmids [61]. Additional helper viruses based on pDG, with its original AAV2 cap genes replaced by cap genes from AAV1 and AAV3–6, were engineered by the same group, in order to achieve increased efficacy in varied cell types [62].
Other innovations have been developed for maximized AAV growth and scalability of production. “Producer” stable cell lines, such as the AAV293 cell line, provide Ad genes to support AAV growth and produce higher viral titers (Agilent #24003). AAV293 cells are human embryonic kidney cells derived from the commonly used HEK293 cell line that have been transformed by sheared adenovirus type 5 DNA. AAV293 cells produce the adenovirus E1 gene in trans, allowing the production of infectious AAV particles when cells are cotransfected with three AAV helper-free system plasmids (an ITR-containing plasmid, pAAV-RC which supplies rep and cap genes in trans, and an E1-deleted helper plasmid). Another producer cell line, 84-31 cells (Human Applications Laboratory, University of Pennsylvania), are a subclone of HEK293 cells that stably express E4 of the adenovirus genome and are used in the same manner as AAV293 [37]. Production technologies have recently been improved to allow production methods that are more scalable than using adherent HEK293 cells for rAAV production. These include HEK293 cell lines that can grow in serum-free suspension, the dual rHSV infection system of suspension baby hamster kidney cells, and the baculovirus expression vector system (BEVS) utilizing Spodoptera frugiperda 9 (Sf9) insect cells (see ref. 28). These systems are especially appropriate for clinical applications, where scalability of rAAV production is essential.
In addition to the preparation of rAAV in large quantities, it must also be purified in large quantities for clinical use. Purification rids the preparation of contaminants such as “empty shells” (AAV particles lacking viral nucleic acid) or adenoviral particles if adenovirus is used as a helper virus. The traditionally used method of sedimentation through CsCl gradients is not scalable and has been replaced by nonionic iodixanol gradient purification or ammonium sulfate precipitation, followed by ion exchange or heparin/agarose column chromatography [63]. This method is based on the finding that AAV2 binds to the heparin sulfate proteoglycan surface molecule [64]. AAV6 is also known to bind heparin with moderate affinity, but despite its high sequence homology with AAV1 (~99.2 %), AAV1 does not exhibit this quality [65] and must therefore be purified using other methods such as ion exchange chromatography [63]. Wu et al. identified a single amino acid (K531) that is essential for conferring the heparin binding characteristics of AAV6 and that introduction of an E531K change in AAV1 yields a heparin binding ability similar to that of AAV6 [30].
1.4 Gene Delivery to the Central Nervous System
There are many potential risks associated with viral gene delivery in humans, and therefore certain considerations must be made for studies involving CNS gene delivery. Unless AAV9 is used in neonates, therapeutic vectors must be administered directly into the CNS. This requires bilateral stereotaxic surgery on anesthetized subjects, which can lead to significant side effects [66, 67]. In this case, gene transfer must be locally restricted to minimize the risks of serious adverse effects that can result from broad distribution. Thus, intracranial injections must be optimized, and vectors must be designed in order to maximize efficiency and specificity of transduction to minimize required dose. Though the first rAAV clinical trials used AAV2, most recent clinical trials use AAV2 ITRs pseudotyped with capsids of other serotypes such as AAV5 or 8 [49, 68, 69], allowing increased transduction efficiency of targeted cell types, as well as avoidance of AAV2-neutralizing antibodies. Increased transduction efficiency is important for minimizing dose.
Although simple injection methods using a constant flow rate have proven to work well for rAAV delivery, some researchers have developed methods that may improve brain delivery of rAAV. For example, convection-enhanced delivery (CED) is a method developed specifically for CNS delivery of large compounds that are not BBB-permeable, in which a constant pressure gradient is applied to the infusate to cause bulk flow, rather than diffusion, through the interstitium [70, 71]. Step cannulae may also be used for injection, which prevent reflux into the track of injection [72]. If a study is designed for widespread delivery to the CNS, therapeutic genes may be delivered via intracerebroventricular or peripheral methods. AAV9, as it is BBB-permeable, may be delivered intravenously in the periphery, avoiding the potential hazards and difficulties of intracranial injection. However, adult animals may show a low neuronal versus glial transduction efficiency with AAV9, and significant amounts of peripheral tissue may be transduced when vector is delivered peripherally [41]. Other delivery methods include targeting brain areas via retrograde transport from muscle to spinal neurons [73]. In certain diseases or disease states, such as stroke, timing of therapy may be crucial for outcome. In AD or other slowly progressing neurodegenerative diseases, therapy may be effective at multiple disease stages.
1.5 Animal Models of Alzheimer’s Disease
The use of transgenic mouse models that recapitulate behavioral and pathological aspects of AD has proven an invaluable tool for studying the disease etiology and effectiveness of therapeutics. AD, the most prevalent neurodegenerative disorder in the world, is characterized by amyloid-β (Aβ) plaques and neurofibrillary tangles, which are thought to cause toxicity responsible for synapse loss and neurodegeneration leading to deficits in memory and cognitive function. Aβ immunotherapies, which have been the main focus of therapeutic development, have shown improvements in amyloid clearance and spatial learning in mouse models expressing mutant APP [74, 75], but these benefits have not yet translated to humans in clinical trials. Recently, a phase III trial of Solanezumab, Eli Lilly and Company’s humanized monoclonal Aβ antibody, reveals small but significant improvements in cognition in mild AD patients [76]. However, these changes are very small and require further analyses.
Despite the disappointing lack of translation of effect from mice to humans of Aβ antibody therapies, animal models remain useful for easily exploring many features of the disease and the extent to which genetic insults affect disease. The first report of a transgenic mouse displaying AD pathology was that of the PDAPP mouse in 1995, which overexpresses mutant Aβ precursor protein (APP) leading to Aβ plaque deposition in relevant brain areas [77]. Tg2576, another line expressing APP Swedish (APPSwe) mutant developed soon after, shows similar pathology as well as age-related cognitive decline [78]. Transgenic mice expressing familial AD-linked mutants of APP and presenilin-1 (PS1) (APP/PS1 mice) have also proven valuable tools in AD investigations, as transgene expression leads to AD-like pathology and phenotype including hippocampal and cortical Aβ deposits and deficits in spatial memory. This spatial memory deficit makes these mice especially useful for spatial memory testing, such as in the radial arm water maze (RAWM) test.
Although the first AD mouse models focused on amyloid pathology, some recent studies have focused on tau models. Mice expressing human mutant tau, such as the P301S mutation, which causes frontotemporal dementia with Parkinsonism linked to chromosome 17 (FDTP-17) in humans, show AD-like tau pathology including hyperphosphorylated tau and neurofibrillary tangles [79]. Recently, Hyman and colleagues developed a transgenic mouse which selectively expresses P301L tau mutant (P301L), associated with FTDP-17, in a subset of neurons in layer II of the entorhinal cortex (EC II) where tau pathology is first seen in AD patients. This allowed them to assess the dissemination of tau and its contribution to subsequent degeneration of critical neural circuits, thought to lead to the deficits in cognitive function and memory seen in AD [80]. This animal should prove useful as an early model of AD and could help in development of therapeutic interventions to ameliorate disease progression. Similarly, our lab has recently developed a rapid tau dissemination model in which AAV6-P301L tau is injected into the medial entorhinal cortex (MEC) of mouse brain, which allows assessment of spread as well as critical cell types and factors involved (manuscript in preparation). These vectors express the tau transgene under the control of a synapsin-1 promoter, which leads to neuron-specific expression. To assess the roles and interaction of Aβ and tau, triple transgenic mice expressing mutations in APP, presenilin 2 (PS2), and P301L tau have been created, and it was suggested that as compared to the P301L mutation alone, Aβ accumulation in these mice led to increased phosphorylation of tau at specific PHF-related residues [81]. Altogether, each mouse model shows unique characteristics of AD, although there is yet a mouse model to show all pathological, phenotypic, and behavioral aspects of the disease. The choice of mouse model for a study should depend on specific aims of the study and how investigators wish to measure therapeutic outcomes.
1.6 Assessing the Outcomes of AD Gene Therapy: Behavioral Testing and Neurophysiology
AD leads to cognitive deficits including learning and memory dysfunction, which correlate with synapse loss and widespread neuron degeneration particularly in the hippocampus [82, 83]. Relevant measures of outcome of AAV-based AD gene therapies in experimental animals thus include spatial memory behavioral testing, electrophysiological assessment of neuron function, and histological analysis of neuron and synapse morphology.
To measure changes in behavior after AD gene therapy, spatial memory tests are often used, one of which is the RAWM test. The RAWM measures the ability of a mouse or rat to remember the location of a hidden underwater platform in repeated trials. Double transgenic APP/PS1 mice show spatial memory deficit as measured by this test [84–86]. Changes in function at the synaptic level can also be measured by electrophysiology. Young PDAPP (J20) and PDAPP (J9) mice, which contain human APP carrying familial AD Swedish (K670N/M671L) and Indiana (V717F) mutations, show a deficit in hippocampal basal synaptic transmission prior to the formation of Aβ plaques, as measured by change in excitatory postsynaptic potential (EPSP) slope [87]. Similarly, Chapman et al. showed that aged APP Swe mice display normal fast synaptic transmission and short-term plasticity, but are impaired in long-term potentiation (LTP) in CA1 and the dentate gyrus [88]. Prominent hippocampal neuronal loss is seen in AD patients, especially in CA1 [82]; thus, measures of synaptic transmission in this region may reveal the efficacy of a certain treatment for AD. Basal synaptic transmission, LTP, and long-term depression (LTD) are common electrophysiological measures of synaptic function and plasticity that can easily be applied to AD studies using AAV gene therapy.
Although widespread neuronal loss is seen in AD, it is believed to be a disease of synaptic failure. This synaptic failure, thought to be caused by the toxicity of amyloid plaques and neurofibrillary tangles, correlates with cognitive decline and is believed to be the initial cause of memory impairment prior to neuron loss [89]. This is supported by electrophysiological studies as outlined previously [87, 88] as well as by studies of synapse density and expression of synaptic markers. Whereas Aβ load and neuron death do not correlate with cognitive decline [90], loss of the presynaptic vesicle protein synaptophysin seen in AD patients in the hippocampus [83] and prefrontal cortex [90] correlates with cognitive decline. Histological examination of the brain readily reveals changes in synapse morphology and density that correlate with cognitive decline in AD. Synapse density can be measured by immunohistochemistry using antibodies against synaptophysin or other synaptic proteins. Hsia et al. used this method to show that PDAPP (J9) mice show significantly decreased synaptophysin-positive terminals in the CA1 region of the hippocampus at as early as 2–3 months of age [87]. Neuronal loss can also be measured with neuronal markers such as microtubule-associated protein 2 (MAP2) or β-3 tubulin.
Increasing evidence shows that adult neurogenesis in the neurogenic regions (subventricular zone and subgranular zone of the dentate gyrus) may be dysregulated in AD, which may be linked to age-dependent memory loss [91, 92]. APP/PS1 mice also show a significant inhibition of neurogenesis [86, 93, 94]. Neurogenesis may be assessed using markers such as doublecortin (Dcx, a marker of newly generated neurons), nestin (a marker of neuronal precursor cells), and bromodeoxyuridine, which is incorporated into newly born cells. Thus, with behavioral, electrophysiological, and histological examination, outcomes of AAV gene therapy for AD can be thoroughly assessed.
1.7 Current AAV Therapies for Alzheimer’s Disease in Animal Studies and Clinical Trials
To date, 80 clinical trials utilizing AAV gene therapy strategies in humans have been completed, are open, or have been reviewed, 17 % of which are for neurological diseases, and two of which are trials for AD therapies [28] (Gene Therapy Clinical Trials Worldwide Database http://www.wiley.com/legacy/wileychi/genmed/clinical/).
Most preclinical and clinical studies for AD gene therapy so far have used neurotrophic growth factors, including nerve growth factor (NGF), fibroblast growth factor 2 (FGF2), and brain-derived neurotrophic growth factor (BDNF) to counteract inhibition of neurogenesis. The first gene therapy used in AD patients tested ex vivo gene delivery of human β-NGF, which has been shown to prevent cholinergic neuron degeneration [95], with primary fibroblasts transduced using retroviral vectors. This phase I trial, completed in 2003, involved forebrain implantation of autologous fibroblasts in eight mild AD patients [66] (ClinicalTrials.gov identifier: NCT00017940). Although two patients were excluded from the trial due to movement during stereotaxic injection, a 22-month follow-up in the six remaining subjects revealed no long-term adverse effects of NGF, an improvement in the rate of cognitive decline (as measured by the Mini-Mental Status Examination and Alzheimer Disease Assessment Scale-Cognitive subcomponent), while serial PET scans showed significant increases in cortical glucose uptake function (as measured by the glucose analog 18-fluorodeoxyglucose) after treatment. Brain autopsy from one subject suggested robust growth responses to NGF.
The two AD gene therapy clinical trials utilizing AAV also tested intracranial delivery of NGF using AAV2 (AAV2-NGF a.k.a. CERE-110; Ceregene, Inc.) [67]. Initial animal studies showed that AAV-mediated NGF expression in the rat basal forebrain (AAV2-NGF; [96]) or medial septum (AAV2/5-NGF) [68] leads to neuroprotection from cholinergic neuron axotomy. A phase I trial involving delivery of AAV2-NGF (CERE-110) to ten patients with mild to moderate AD was completed in 2010 (ClinicalTrials.gov identifier: NCT00087789) [97], and 50 patients are now recruited for a CERE-110 phase II trial (ClinicalTrials.gov identifier: NCT00876863). The open-label phase I study shows that AAV-NGF is safe and well-tolerated for up to 2 years and that the recombinant NGF is expressed in transduced cells and maintains bioactivity [98].
BDNF has also shown promise as a gene therapy treatment for AD. In preclinical animal studies, Tuszynski and colleagues delivered BDNF via a lentiviral vector to the entorhinal cortex of APP-transgenic mice (Swedish and Indiana APP mutations) and aged rats and finally to nonhuman primates modeling entorhinal cortex neuronal death induced through bilateral radiofrequency lesions to the perforant path, which connects the entorhinal cortex to the hippocampus [99]. In rodents, they reported restoration of spatial memory deficits in both models, but no effect on neuronal number or Aβ plaque density. BDNF treatment partially normalized changes in gene expression implicated in AD and aging, in the mouse and rat model, respectively. In the primates, BDNF injections (lentivirus-BDNF-GFP) given 4 days prior to lesioning prevented cell death and improved monkeys’ performance in a spatial discrimination task.
FGF2, an established neurogenic factor of proliferation and differentiation for multipotent neural progenitors [100–102], is additionally under investigation for AD gene therapy. FGF2 is expressed in neurogenic regions in the CNS and has been implicated in the control of adult neurogenesis because of its effect on proliferation and fate choice of adult neural progenitor cells [103, 104]. Our lab has recently shown that bilateral delivery of FGF2 to the hippocampi of APP/PS-1 bigenic mice using an AAV2/1 hybrid (AAV2/1-FGF2) results in significantly improved spatial learning in the radial arm water maze test; enhanced neurogenesis as measured by number of doublecortin, BrdU/NeuN, and c-fos-positive cells in the dentate gyrus; and enhanced clearance of fibrillar Aβ in the hippocampus. AAV2/1-FGF2 injection was also shown to enhance long-term potentiation in another APP mouse model (J20) compared with control AAV2/1-GFP-injected littermates [105].
While growth factors have shown beneficial effects as gene therapies for AD, our lab has also shown that AAV-mediated delivery of certain anti-inflammatory chemokine and cytokine genes may counteract the variety of inflammatory processes involved in AD progression. AAV2/1-mediated delivery of the anti-inflammatory cytokine interleukin-4 (IL-4) into the hippocampus results in sustained expression of IL-4, reduced astro-/microgliosis, Aβ oligomerization and deposition, enhanced neurogenesis, and improved spatial learning [94]. Similarly, AAV2/1-mediated hippocampal neuronal expression of the mouse anti-inflammatory cytokine IL-10 gene ameliorats cognitive dysfunction and was associated with reduced astro-/microgliosis, enhanced plasma Aβ levels, enhanced neurogenesis, and improved spatial learning in APP/PS-1 mice [86]. Along the same lines, suppressing expression of the pro-inflammatory chemokine CCL2 via AAV2/1-mediated delivery of 7ND, a dominant-negative CCL2 mutant, in APP/PS-1 mice reduced astro-/microgliosis, β-amyloidosis, and improved spatial learning [85]. Whether delivering growth factors to reverse neurodegeneration and promote neurogenesis, or cytokines and chemokines to counteract harmful neuroinflammation in AD, these studies highlight the AAV2/1 system as a useful tool for CNS gene delivery. In this chapter, we describe AAV2/1-mediated gene delivery to the CNS, from rAAV construction to injection and assessment of gene expression. We provide detailed methods for construction of AAV2-MCS-WPRE expression vectors carrying the gene of interest, generation of adenovirus-free hybrid AAV2/1 vectors through cotransfection of helper plasmid, AAV1 and AAV2 constructs into AAV293 cells, purification and tittering of rAAV, stereotaxic injection into animals, and finally histological analysis of gene expression.
2 Materials
1.
For all tissue culture procedures, use standard tissue culture incubator, with 5 % CO2 humidified environment at 37 °C. A separate 37 °C incubator should be used for incubation of bacteria, to avoid contamination.
2.
The use of AAV-293 cells is highly recommended for viral generation. The cells are derived from HEK293 cell line and produce higher viral titers. AAV particles are produced by transfection of the cells with three plasmids (an inverted terminal repeat (ITR) sequences-containing plasmid, AAV replication and capsid gene-containing plasmid, the early region 1 (E1)-deleted adenoviral helper plasmid). The E1 gene is provided by the AAV-293 cells in trans to produce infectious AAV particles, and viral generation should be performed in a biosafety level 2 (BSL-2)-approved facility. Here we use a pAAV serotype 2 including cytomegalovirus/chicken β-actin hybrid promoter, multicloning sites and Woodchuck posttranscriptional regulatory element (pAAV2-MCS-WPRE), p5E18RXC1, and pAd∆F6 (both provided from Dr. James Wilson, the University of Pennsylvania Gene Therapy Program).
3.
In Tables 2 and 3, we provide a list of all reagents and solutions described in the Sect. 3.
Table 2
Consumables
Item | Supplier | Item no. |
---|---|---|
1. Generation of rAAV | ||
1.1 Subcloning | ||
p5E18RXC1 (AAV1 plasmid) | Dr. James Wilson, Vector Core, University of Pennsylvania | |
pAd∆F6 helper plasmid | Dr. James Wilson, Vector Core, University of Pennsylvania | |
DH5α competent cells | Invitrogen | 18258-012 |
LB Broth Base, Powder | Invitrogen | 12780-029 |
Ampicillin | Invitrogen | 11593027 |
Qiagen Miniprep Kit | Qiagen | 27104 |
Qiagen Endo-Free Maxi Kit | Qiagen | 12362 |
2. rAAV purification | ||
2.1 Transfection into AAV293 cells | ||
AAV-293 cells | Agilent | 240073 |
T150 cell culture flasks | Sigma-Aldrich | CLS3291 |
50 ml conical tubes | BD Biosciences | 352070 |
Cell scraper | Sigma-Aldrich | CLS3009 |
2.2 Crude AAV particle extraction | ||
Benzonase nuclease | Millipore | 70664 |
2.3 Iodixanol purification | ||
Iodixanol (OptiPrep) 60 % w/v solution (250 ml) | Sigma-Aldrich | D1556 |
Quick-seal tubes (25 × 89 mm) | Beckman-Coulter | 342414 |
10 ml syringe | Fisher Scientific | 01-911-233 |
18 G needle | BD Biosciences | 305833 |
2.4 HiTrap Q HP column chromatography and concentration | ||
Hitrap heparin HP (5 × 1 ml) | GE Healthcare | 17-0406-01 |
Centriprep Ultracel-30 | Millipore | 4306 |
2.5 AAV tittering | ||
2.5.1 Viral DNA extraction | ||
1.5 ml Eppendorf tubes | Eppendorf | 22364111 |
DNase 1 | Promega | M6101 |
20 mg/ml proteinase K | New England Biosciences | P8102S |
Phenol/chloroform/isoamyl alcohol 25:24:1 | Sigma | P2069 |
Nuclease-free H2O | Invitrogen | AM9935 |
2.5.2 Calculation of physical titer | ||
Amicon Centriprep Ultracel-100 | Millipore | 4305 |
3. Delivery to mouse brain | ||
10 ml Hamilton syringe | Hamilton | 201350 |
Ketamine | ||
Xylazine | ||
Small cordless drill | Roboz | RS-6300 |
Stereotaxic apparatus | Harvard Apparatus | 726049 |
4. Analysis of brain expression | ||
4.1 Analysis of gene expression, neurogenesis, and amyloid pathology | ||
BrdU antibody | University of Iowa Hybridoma Bank | G3G4 |
Doublecortin antibody | Santa Cruz | sc-8066 |
Thioflavin-S | Sigma-Aldrich | T1892 |
DAB + Chromogen EnVision™ + system/HRP, mouse (DAB+) | Dako | K4007 |
Amyloid beta-peptide rabbit polyclonal antibody | Invitrogen | 71-5800 |
Table 3
Reagents
Reagent | Volume (ml) | Component | Final concentration |
---|---|---|---|
2.1 Transfection into AAV-293 cells | |||
Complete DMEM (cDMEM) (1 l) | 880 | DMEM (Invitrogen, 12491-015) | |
100 | Fetal bovine serum | 10 % | |
10 | Penicillin/streptomycin | 100 U/ml and100 μg/ml | |
10 | l-glutamine | 2 mM | |
2× HEPES-buffered saline (HBS) | NaCl | 280 mM | |
HEPES | 50 mM | ||
Na2HPO4 | 1.5 mM | ||
pH 7.12 | |||
2.2 Crude AAV particle extraction | |||
Cell suspension buffer | Tris–HCl, pH 8.5 | 50 mM | |
NaCl | 150 mM | ||
dH2O to volume | |||
2.3 Iodixanol gradient purification | |||
PBS-MK (1 l) | 100 | 10 × PBS | 1× |
2.0 | 0.5 M MgCl2 | 1 mM | |
5.0 | 1 M KCl | 5 mM | |
893 | ddH2O | ||
2.4 HiTrap Q HP column chromatography and concentration | |||
Buffer W (wash buffer) | Tris–HCl, pH 8.5 | 20 mM | |
NaCl | 15 mM | ||
ddH2O to volume | |||
Buffer E (elution buffer) | Tris–HCl, pH 8.5 | 20 mM | |
NaCl | 500 mM | ||
ddH2O to volume |
3 Methods
3.1 Generation of rAAV Vectors
Recombinant AAV vectors are easily produced by exchanging the whole viral genome except ITRs with any genomic DNA or complementary DNA (cDNA) of interest. However, the DNA with all essential elements for AAV generation must be less than 5.0 kb in length because of the viral packaging limit. A standard procedure of DNA subcloning is sufficient for this purpose. Here we use a pAAV2-MCS-WPRE as a cis-plasmid for AAV generation.
3.1.1 Subcloning of Genome of Interest into pAAV2-MCS-WPRE
1.
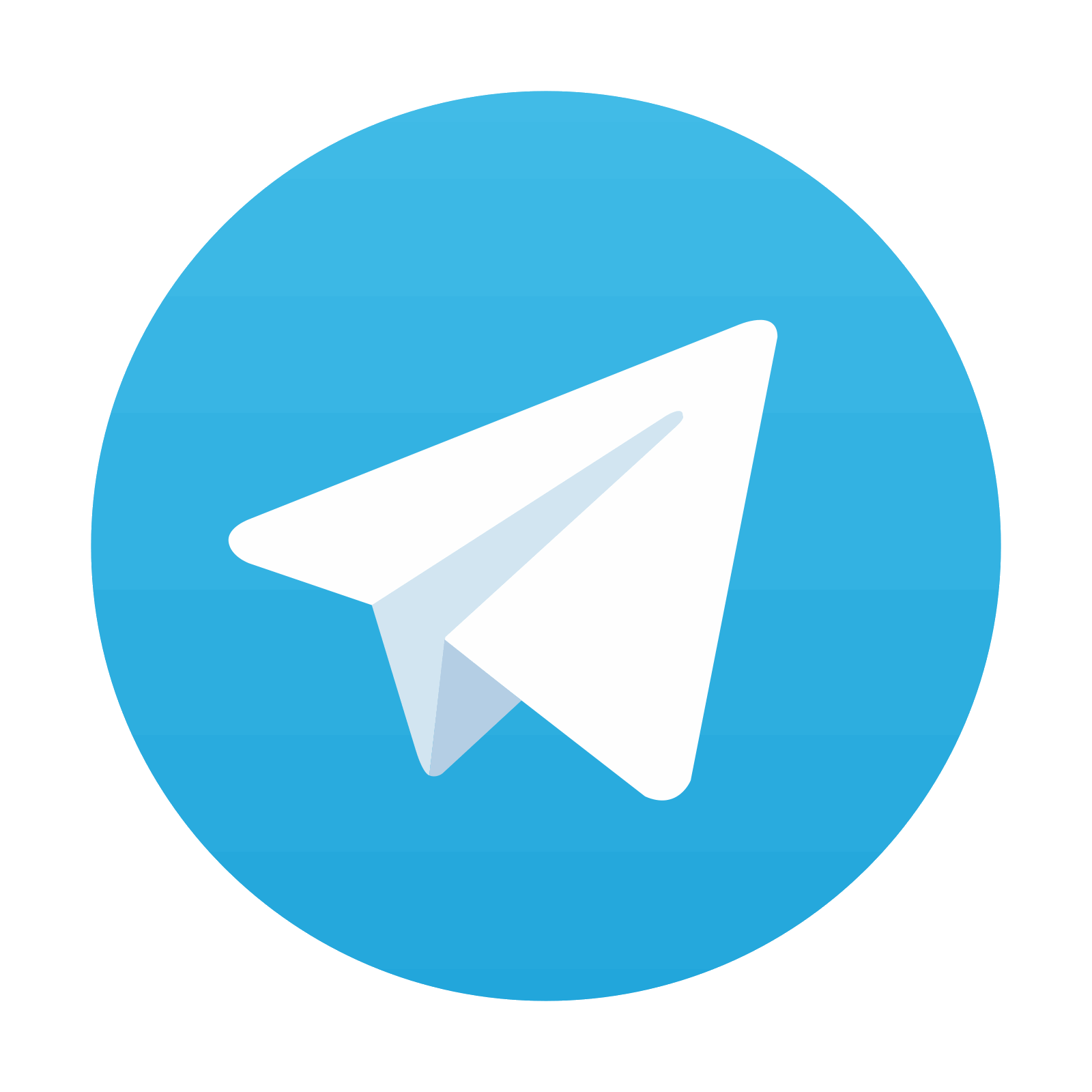
Obtain DNA fragments to be inserted into MCS of pAAV2-MCS-WPRE containing ITRs, which will flank the gene of interest. The DNA can be a proofreading PCR amplification of complementary DNA region or directly dissected from other plasmids including cDNAs.
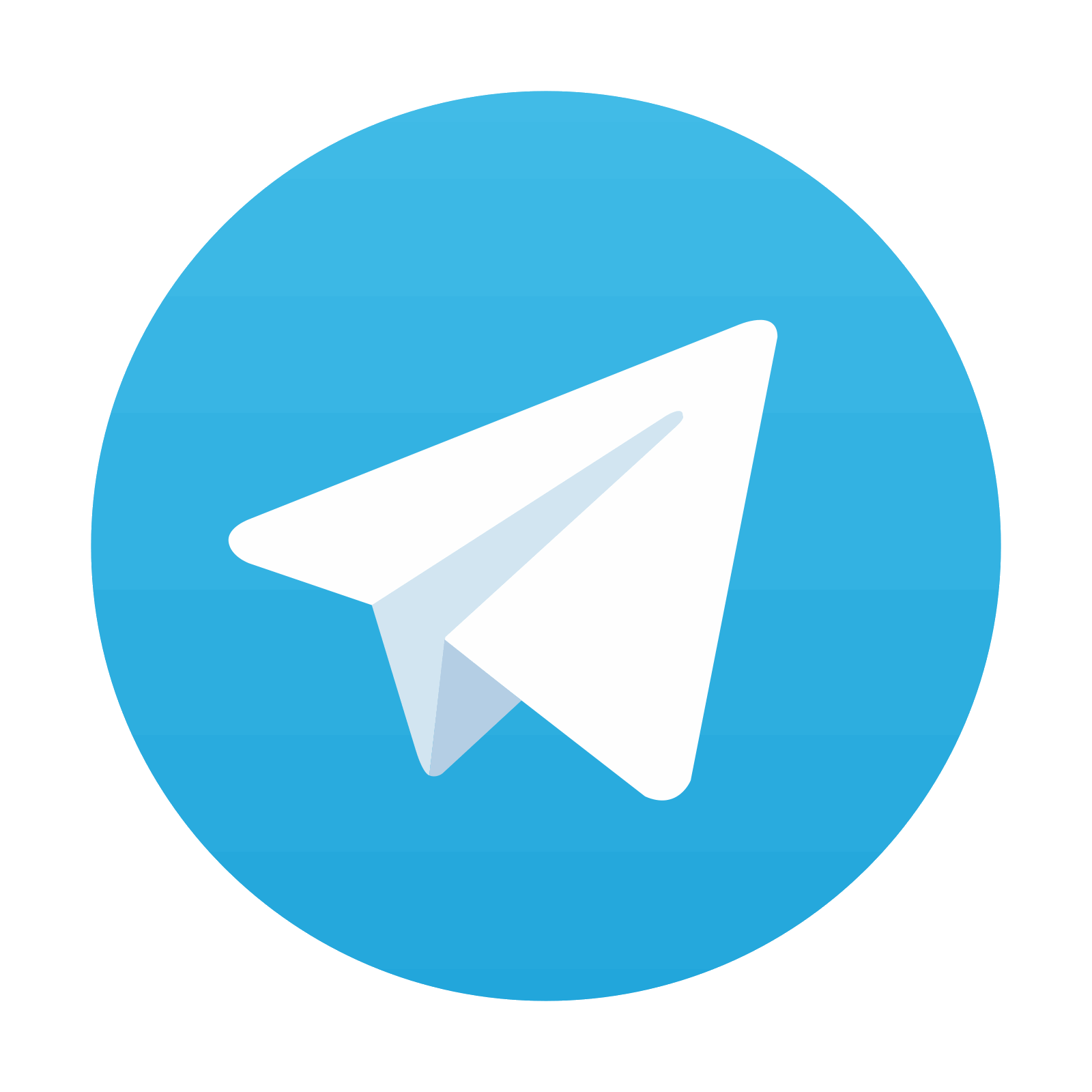
Stay updated, free articles. Join our Telegram channel
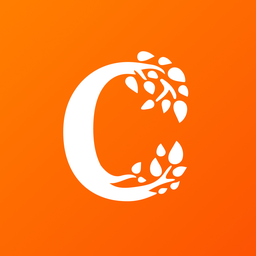
Full access? Get Clinical Tree
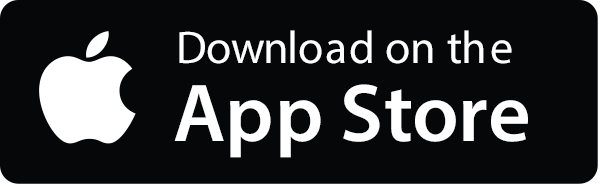
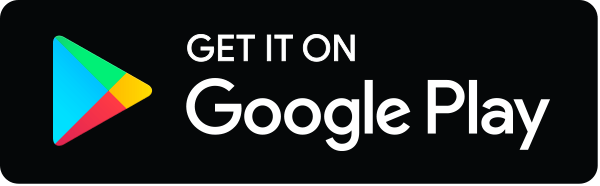
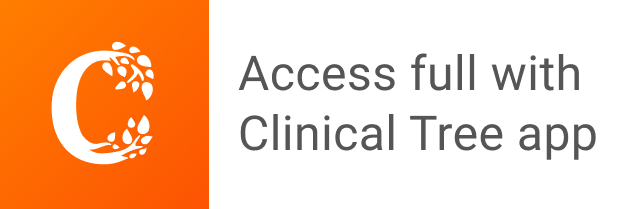