Abstract
Amyotrophic lateral sclerosis (ALS) is a disease with a poorly understood etiology. There are a wide variety of abnormalities present, including mitochondrial dysfunction, glutamate excitotoxicity, oxidative stress, astrogliosis and microgliosis, loss of innervation of neuromuscular junctions, and death of motor neurons. However, the relative contribution of these factors to disease initiation and progression is unclear at best, making it difficult to know which one or ones to focus on. Therefore, a wide variety of approaches have been investigated to treat this disorder, including gene therapy, cell therapy, and novel drugs. This chapter will focus on the therapeutic transgenes that have been studied to date. These transgenes range from those that address a single abnormality, such as apoptosis, to those that are expected to treat several defects simultaneously. While this range of approaches has demonstrated some improvement in the ALS phenotype in animal models, none has become the silver bullet that will halt ALS in its tracks.
Keywords
Amyotrophic lateral sclerosis, gene therapy, transgenes, neurotrophic factors, apoptosis, glutamate excitotoxicity
Outline
Introduction 168
Antiapoptosis 168
Glutamate Signaling (Glutamate Transporter 1, ADAR2/AMPA) 172
Antioxidant Genes 175
Neurotrophic Factors 177
Cardiotrophin-1 178
Granulocyte-Colony Stimulating Factor 179
Vascular Endothelial Growth Factor 180
Brain-Derived Neurotrophic Factor 183
Glial-Derived Neurotrophic Factor 183
Insulin-Like Growth Factor 1 186
Hepatocyte Growth Factor 188
Combination Therapies 188
Tetanus Toxin heavy Chain C-Fragment 190
Angiogenin 191
Single-Chain Antibodies 191
Possible Future Candidates 192
Timing of Therapy 193
Concluding Remarks 194
References
Introduction
As discussed in Chapter 4 , Molecular Mechanisms of Amyotrophic Lateral Sclerosis, amyotrophic lateral sclerosis (ALS) is a disease with a poorly understood etiology. There are a wide variety of abnormalities present, including mitochondrial dysfunction, glutamate excitotoxicity, oxidative stress, astrogliosis and microgliosis, loss of innervation of neuromuscular junctions (NMJs), and death of motor neurons (MNs). However, the relative contribution of these factors to disease initiation and progression is unclear at best. Therefore, a wide variety of approaches have been investigated to treat this disorder. This chapter will focus on the therapeutic transgenes that have been studied to date. These transgenes range from those that address a single abnormality, such as apoptosis, to those that are expected to alter several defects simultaneously. While this range of approaches has produced improvements in the ALS phenotype in animal models, none has become the silver bullet that will halt ALS in its tracks.
Antiapoptosis
One of the defining characteristics of ALS is the loss of upper and lower MNs. Since the death of these cells could be a direct cause of NMJ denervation, one obvious therapeutic approach would be to prevent cell death from occurring. Cells can be killed by a number of mechanisms ranging from a tidy programmed cell death, where the cell essentially breaks itself down to be phagocytized by nearby cells, to messy necrosis, where the cell spills its contents into the extracellular space, inducing inflammation. It is generally believed that MN death occurs via a process of programmed cell death known as apoptosis, or at the very least, something very similar to it.
Apoptosis was first described by Kerr et al. in 1972, and it involves a highly regulated series of steps ( Fig. 8.1 ). There are three main pathways in apoptosis: death receptor-mediated, mitochondrial-mediated, and endoplasmic reticulum-mediated. Based on cell culture models, animal models, and postmortem tissue from ALS patients, it appears that the mitochondrial-mediated pathway is likely the most relevant to MN death in this disease. In this pathway, the action of proteins like Bcl-2-associated X (Bax) and Bcl-2-homologous antagonist/killer (Bak) cause the mitochondria to release cytochrome c and other factors into the cytoplasm. This in turn activates a cascade of caspases, including caspases 3 and 9. This pathway is inhibited by a number of proteins, including X-linked inhibitors of apoptosis protein (XIAP), B-cell lymphoma 2 (Bcl-2), and B-cell lymphoma extra large (Bcl-X L ).

During apoptosis, caspases break down the proteins of the cytoskeleton, causing cells to shrink and round. The nuclear chromatic condenses and endonucleases are activated, fragmenting the DNA. Finally, the plasma membrane blebs, breaking the cells into a host of small vesicles that are phagocytosed by macrophages. Importantly, in contrast to necrotic cell death, the contents of the cytoplasm are not spilled into the extracellular space. Thus, apoptosis does not activate inflammatory pathways through intracellular milieu release.
There are a number of signs that apoptosis occurs in both animal models of ALS and ALS patients. In mice expressing mutant SOD1 (SOD1 mice), the antiapoptotic Bcl-2 and Bcl-X L proteins exhibit reduced expression, while the proapoptotic proteins Bcl-2-associated death protein (Bad) and Bax are upregulated during the symptomatic phase of the disease. Knocking out Bax in this model completely blocks MN death. Cytoplasmic cytochrome c levels increase in the spinal cords of SOD1 mice as they age, peaking when the mice reach the early symptomatic stage of the disease. Caspase 1 and caspase 3 mRNAs are also both upregulated, first in neurons and then in glial cells. Sequential activation of several downstream caspases is also observed.
In ALS patients, a similar pattern emerges. Bax and Bak are enriched in the mitochondrial membrane fraction, while Bcl-2 is decreased in this compartment. In addition, the binding of Bcl-2 to Bax, necessary for the suppression of Bax function, is also significantly reduced. Similar to the animal model, ALS patients also exhibit elevated activity of caspase 1 and caspase 9.
A number of studies have demonstrated that inhibiting apoptosis can slow or prevent some aspects of ALS. Inhibiting caspase activity is one potential approach. Overexpression of XIAP in neurons in a SOD1 mouse reduced caspase activity and extended life span. Intracerebroventricular infusion of N -benzyloxycarbonyl-Val-Ala-Asp-fluoromethylketone (zVAD-fmk), a broad-spectrum caspase inhibitor, significantly extended the life span of the SOD1 mouse when delivered by an osmotic pump beginning at 60 days of age. Disease onset was delayed by 20 days and survival was increased by 27 days. MN death was reduced by the greatest amount in the cervical spinal cord, possibly suggesting poor delivery of the drug to the lumbar cord. It should be noted that this therapy significantly reduced the production of mature interleukin (IL)-1β, a proinflammatory factor. Thus, part of the effect of this treatment could be due to dampening of inflammation.
Rather than targeting later steps in apoptosis, it may be more effective to target the initiating events. Overexpression of Bcl-2 in transgenic mice had previously been shown to block apoptosis due to a variety of factors, including the culling of excess neurons during normal development. This protein was also neuroprotective in other models of neuron death, including ischemia and axotomy.
To determine whether overexpression of Bcl-2 could impact ALS, transgenic mice expressing Bcl-2 from the neuron-specific enolase (NSE) promoter were crossed with SOD1-G93A mice to create double transgenics (Bcl-2;SOD1). When compared to SOD1 mice, Bcl-2;SOD1 mice exhibited a 33-day delay in disease onset, and life span was increased by 35 days. MN loss was also delayed, but end-stage animals in both groups had similar levels of MN death. The loss of NMJ innervation and axons in the phrenic nerve exhibited a similar pattern. Thus, Bcl-2 delayed, but could not prevent, MN loss. While Bcl-2 has a critical role in apoptosis, the authors suggested that the effects that they observed may not simply be due to a direct effect on apoptosis. The Bcl-2;SOD1 mice also showed smaller numbers of ubiquitin-positive MNs relative to age-matched SOD1 mice, something unexpected if Bcl-2 was only affecting apoptosis. The authors speculate that antioxidant properties of Bcl-2 could also be contributing to the delayed disease onset. It may be important to note that, while Bcl-2 can protect neurons from death, it may not prevent axon die back, a process that is critical in ALS.
Another member of the Bcl-2 family of proteins with antiapoptotic activity is Bcl-X L . When tested in a tissue culture model of glutamate-induced cytotoxicity, overexpression of Bcl-X L substantially reduced TUNEL positivity and increased cell viability in primary MNs. Ohta et al. studied a recombinant protein consisting of Bcl-X L fused to the protein transduction domain (PTD) of the HIV TAT protein. The PTD is of interest because it can allow the coupled protein to cross cellular membranes, including those of the blood–brain barrier. Thus, one can use intravenous, intraperitoneal, or intrathecal delivery to get a recombinant protein into the cytoplasm of cells. When injected intraperitoneally in an ischemic brain injury model, this fusion protein (TAT-Bcl-X L ) was able to reduce caspase-3 activation, substantially inhibit DNA fragmentation, and reduce neurological deficits. In SOD1 mice, TAT-Bcl-X L was delivered by intrathecal infusion over a period of 28 days, beginning at 91 days of age. This therapy delayed disease onset by 10 days and increased life span by 13 days. Motor function showed improvement, and MN death was reduced relative to controls. Markers of apoptosis, including caspase 3 and caspase 9, activation and TUNEL positivity were also significantly reduced.
One other study should also be noted here. Kaspar et al. examined the effects of exercise in SOD1 mice. Ad libitum exercise increased life span by 24 days. Interestingly, exercise also elevated the expression of the antiapoptotic genes Bcl-2 and Bcl-X L , suggesting that exercise could function, in part, by increasing MN resistance to apoptosis.
In the early stage of disease progression in SOD1 mice, activation of caspase 1, rather than caspase 8, leads to the cleavage of BH3-interacting domain death agonist (Bid), yielding truncated Bid (tBid), the active form of Bid that can initiate the mitochondrial apoptotic pathway. Thus, targeting caspase 1 is a promising target for an antiapoptotic therapy. Friedlander et al. created a transgenic mouse expressing a dominant negative form of caspase 1 by mutating an active site cysteine residue to glycine. Crossing this mouse with a SOD1 mouse substantially reduced the amount of cytochrome c release from mitochondria and delayed the activation of caspases 3 and 9. The dominant negative caspase 1 did not delay disease onset, but it did significantly increase survival by 21 days.
While there have been many successful studies using transgenes to inhibit apoptosis in animal models of ALS, only one study has evaluated a gene therapy approach. Azzouz et al. injected an adeno-associated virus (AAV) vector expressing Bcl-2 into the lumbar spinal cord of SOD1 mice. The therapy delayed spontaneous fibrillation potentials in the gastrocnemius muscle by approximately 10 days and increased MN survival in the injected region. However, no improvement in life span was detected.
The use of parenchymal injections might explain why the effect of this therapy is so muted. In contrast to the previous studies, Bcl-2 was expressed only in the region injected. Thus, disease progression in the rest of the spinal cord was free to proceed unchecked. In addition, even near the injection sites, it is unlikely that all of the target cells were transduced. A broader delivery of the transgene would likely increase its effectiveness. It would be interesting to see how Bcl-2 would perform using an AAV9 vector, which can provide robust expression throughout the central nervous system (CNS) when delivered intravenously.
Glutamate Signaling (Glutamate Transporter 1, ADAR2/AMPA)
Upper MNs, residing in the motor cortex, communicate with lower MNs in the spinal cord using the excitatory neurotransmitter glutamate. While it is the most prevalent neurotransmitter in the CNS, excess levels of glutamate can be toxic to neurons due to overstimulation of glutamate receptors. This aberrant signaling leads to excessive calcium influx into the cell and, possibly, to activation of other signaling pathways.
Glutamate-induced cytotoxicity is believed to contribute to the demise of MNs in ALS. In the spinal cord, excitatory amino acid transporter 2 (EAAT2, Glt1) is primarily responsible for glutamate clearance. Studies of ALS patients have demonstrated abnormally spliced EAAT2 transcripts in the spinal cord, loss of EAAT2 expression, reduced glutamate transport, and increased levels of glutamate in the cerebrospinal fluid, all pointing to a possible role for glutamate-induced excitotoxicity in the development and/or progression of ALS. Thus, increasing EAAT2 expression was hoped to help mitigate the disease.
To test this hypothesis, Guo et al. generated transgenic mice that overexpress EAAT2 in astrocytes. When this transgene was crossed with the SOD1 mouse to create EAAT2/SOD1 double transgenics, there was a small delay in the onset of motor decline, but the onset of paralysis was not delayed, and there was no improvement in life span. Interestingly, EAAT2/SOD1 mice did show increased MN survival relative to age-matched SOD1 mice. Astrogliosis appeared to be unaffected, but the double transgenic mice did exhibit lower levels of high-molecular-weight SOD1 aggregates. Similar results were found in a study evaluating an AAV8 vector expressing EAAT2 from the astrocyte-specific promoter Gfa2. Despite transducing 83% of GFAP + cells, no improvement was seen in motor function and MN loss was not reduced. The authors of both studies concluded that the loss of EAAT2 might contribute to, but probably does not cause, MN degeneration.
How glutamate contributes to the progression of ALS may be a more complicated story than a simple model of poor glutamate clearance. Glutamate signaling in MNs primarily occurs through α-amino-3-hydroxy-5-methyl-4-isoxazolepropionic acid (AMPA) receptors. In general, these receptors are heterotetramers, composed of four subunits. Four different AMPA receptor subunits have been described (GluA1–GluA4, also known as GluR1–GluR4). Depending on the cell type and other factors, different subunits are expressed and combine to form AMPA receptors. The GluA2 subunit is particularly interesting. The mRNA encoding this protein is the target of RNA editing by adenosine deaminase acting on RNA 2 (ADAR2). This enzyme induces a posttranscriptional modification, converting adenosine (A) to inosine (I) by deamination. During translation, inosine pairs with tRNAs as if it were guanosine. In the case of GluA2, this results in glutamine-to-arginine substitution in the protein. This change substantially alters the channel of AMPA receptors containing GluA2. When arginine is present (the edited form, GluA2(R)), the channel is permeable to sodium and potassium but not to calcium. However, in the absence of editing (GluA2(Q)), the presence of the glutamine residue also allows calcium to pass through the channel. In addition, the edited form of GluA2 affects assembly in the ER and slows trafficking to the plasma membrane. Thus, the presence of unedited GluA2 can increase conductance both through permitting calcium influx and increasing the number of channels on the cell surface (reviewed in refs and ).
Under normal conditions for most cells, including MNs, the vast majority of GluA2 subunits are of the edited variety. Interestingly, incomplete editing of GluA2 is present in some neurodegenerative disorders, including Huntington’s disease, Alzheimer’s disease, and schizophrenia. In patients with sporadic ALS, a fraction of MNs exhibit loss of expression of edited GluA2. In addition, a subset of spinal MNs from ALS patients lack expression of ADAR2, an abnormality not found in control cases. These ADAR2 − cells exhibit TDP-43-positive inclusions, a common finding in ALS. These observations have led some to suggest that ADAR2 deficiency could lead to the development of TDP-43 inclusions and thus represents a possible therapeutic target. Alternatively, it is equally possible that TDP-43 inclusions lead to loss of ADAR2 expression and GluA2 editing. A number of mouse models have been created to begin testing the former hypothesis.
Kuner et al. investigated mice expressing GluA2 with an asparagine inserted in place of the edited codon (GluA2(N)) in addition to the endogenous GluA2 alleles. Like GluA2(Q), this subunit creates an AMPA receptor that is permeable to calcium. These mice began to show deficits in motor function around 35 weeks of age, although they did not progress to paralysis by 2 years of age. By 1 year of age, there was a statistically significant decrease in the number of neurons in the ventral horns. At 2 years of age, about 30% of these cells had been lost. Crossing the GluA2(Q) mouse with the SOD1 mouse led to a significant increase in disease severity. In contrast, mice overexpressing GluA2(R) in MNs have decreased calcium influx into cells. When crossed with SOD1 mice, disease severity was reduced. These studies point to an important role for calcium influx through AMPA receptors in ALS disease progression.
To directly determine whether the failure to edit GluA2 might contribute to ALS disease progression, Hideyama et al. created a conditional ADAR2 knock-out by crossing a floxed ADAR2 mouse with a mouse expressing Cre recombinase from the vesicular acetylcholine transporter. This scheme significantly reduced ADAR2 expression in MNs and led to a significant fraction of MNs expressing unedited GluA2. This mouse exhibited a progressive loss of strength and motor function, although at a fairly protracted rate. Median life span was 82 weeks. The mice exhibited many symptoms of ALS, including fibrillation potentials and fasciculations, loss of NMJ enervation, astrogliosis and microgliosis, and MN loss. Importantly, crossing this mouse to one expressing a GluA2 mutated to only express the edited allele rescued the phenotype, demonstrating that the abnormalities observed in ADAR2-deficient mice were due to the loss of GluA2 editing.
Given the potential role that ADAR2 loss might play in the development or progression of ALS, Yamashita et al. examined gene therapy in the conditional ADAR2 knock-out mouse. They performed an intravenous injection of an AAV9 vector expressing ADAR2 into these mice before or at disease onset. This therapy significantly increased the amount of GluA2 that was correctly edited. Performance on the rotarod task, which deteriorated over time in untreated mice, stabilized with therapy for at least 20 weeks. At 39 weeks of age, MN survival and axon counts were higher in the treated group relative to controls.
While it is not yet clear whether defects in GluA2 editing represent an early or late event in the progression of ALS, the data from the mouse models strongly suggest that altering calcium influx via modulation of the AMPA receptor can at least slow the progression of ALS. Since reduced ADAR2 expression in MNs seems to be common in sporadic ALS patients, gene therapy to restore its lost function represents a plausible approach to treat this aspect of the disorder. However, given the mild presentation of disease in the conditional knock-out of ADAR2, it is likely that ADAR2 gene therapy would need to be given in the context of additional therapies that target other aspects of ALS.
Antioxidant Genes
Oxidative stress may also contribute to ALS disease progression. Reactive oxygen species (ROS), including hydrogen peroxide and the superoxide radical anion, are generated as a byproduct of aerobic metabolism. Unneutralized ROS can react with a number of molecules. For instance, the superoxide radical anion can combine with a nitric oxide radical, producing peroxynitrite which can go on to react with tyrosine residues producing nitrotyrosine. Other molecular changes include carbonylation and oxidative damage to DNA. A number of signs of oxidative damage have been found in postmortem tissue samples taken from ALS patients. These include higher 3-nitrotyrosine levels, evidence for an increased carbonylation, and elevated oxidative damage to DNA. In an in vitro model of ALS, application of catalase can increase cell viability, suggesting that targeting oxidative stress could be beneficial.
Metallothioneins (MTs) are a set of zinc binding proteins that likely have many functions, including protection from oxidative stress. They can also serve as zinc chaperones for SOD1. When zinc is depleted from SOD1, the enzyme more rapidly catalyzes the nitration reaction. Interestingly, mutant SOD1 also has reduced zinc binding and increased nitration activity, possibly pointing to one mechanism of its toxicity. Taken together, these observations suggest that MTs can play a role in slowing ALS disease progression.
Puttaparthi et al. hypothesized that inhibiting one or more MTs would exacerbate disease progression. Three MTs are expressed in the nervous system of mice: MT-I and -II are expressed in glial cells, while MT-III is expressed in neurons. When MT-I and MT-II were both knocked out in a SOD1 animals (MT-I − ;MT-2 − ;SOD1 mice), mean survival time was reduced by 32 days (229 vs 261 days). Disease onset was approximately 2 months earlier in the MT-I − ;MT-2 − ;SOD1 mice. Astrogliosis was more pronounced at 5.25 months in the MT-I − ;MT-2 − ;SOD1 mice than in SOD1 mice, but MN counts were similar between both groups at all time points evaluated. This suggested the glial component of the disease could be altering the function of the remaining MNs, resulting in a faster decline in motor function than would be predicted from the MN counts. When MT-III was knocked out in SOD1 mice, survival was reduced by 51 days (202 vs 253 days). In contrast to the MT-I/II knockout, disease onset was not significantly affected by the loss of MT-III. Instead, the decline in motor function was substantially steeper. In addition, MN loss was significantly greater at 5.25 months in MT-III knockouts, suggesting that MT-III protects MNs from death. These results suggest that altering the expression of MTs using gene therapy could also slow disease progression.
Hashimoto et al. investigated weekly injection of Ad5-MT-III into female SOD1 mice beginning at 20 weeks of age, just before the animals would become overtly symptomatic. The vector was injected into the lower limbs with the hope of getting retrograde transport of the vector to the MNs. Disease onset was not affected, which is not remarkable since treatment was so close to onset. MN counts were significantly higher at 160 days of age in Ad5-MT-III-treated animals. Life span was longer in treated mice as well. The authors found that the Ad vectors themselves were toxic and could accelerate aspects of the disease, and thus a different delivery system should be investigated.
Two other antioxidant genes have been evaluated in the context of gene therapy. Peroxiredoxin 3 (PRDX3) is a mitochondrial thioredoxin-dependent hydroperoxidase and is upregulated in response to oxidative stress. Nuclear factor erythroid 2-related factor 2 (NRF2) is involved in antioxidant response element-mediated gene expression and regulates many phase 2 detoxifying enzymes. Many of the genes controlled by NRF2 are downregulated in ALS. Overexpression of PRDX3 in NSC34 cells significantly increased cell survival under basal conditions and serum starvation, but did not have a significant effect in the face of oxidative stress induced by menadione. This was attributed to the severity of the menadione treatment and the susceptibility of the cells. Murine NRF2 showed protection against menadione in astrocytes and increased survival of NSC34 cells expressing mutant SOD1. These genes were evaluated in vivo using intramuscular injection of AAV6 vectors into 30-day-old SOD1 mice (facial muscles, tongue, intercostal muscles, diaphragm, and hindlimb). No improvement was seen with either vector. However, at endpoint, only 5% of the surviving neurons in green fluorescent protein controls were positive for the transgene. This suggested that poor transduction was to blame for the lack of efficacy.
Neurotrophic Factors
Neurotrophic factors are a set of signaling molecules that are involved in the development and maintenance of the nervous system. They bind to receptors on the cell surface, activating two major signaling cascades: the phosphoinositol-3 kinase (PI3K)/Akt pathway and the mitogen-activated protein kinase/extracellular signal-regulated kinase (MapK/Erk) pathway. In addition, IL-6-related cytokines and granulocyte-colony stimulating factor (G-CSF) can also activate the Janus kinase/signal transducer and activator of transcription (Jak/Stat) pathway ( Fig. 8.2 ). Since space does not permit a detailed description of the biology of each factor, the reader is directed to several excellent reviews: insulin-like growth factor 1 (IGF-1), vascular endothelial growth factor (VEGF), brain-derived neurotrophic factor, glial-derived neurotrophic factor (GDNF), G-CSF, and the IL-6 family of cytokines.

These signaling cascades allow neurotrophic factors to act on the ALS disease state in a number of ways. All three pathways play important roles in regulating axon growth and regeneration, maintaining and, possibly, restoring NMJ innervation. They can also block apoptosis by inhibiting proapoptotic factors and upregulating antiapoptotic factors. Activated Akt phosphorylates serine residues on Bad, caspase 9, and some forkhead family transcription factors, inactivating these proteins and inhibiting apoptosis. The Erk pathway similarly phosphorylates Bad, Bcl-2-interacting mediator of cell death (Bim), and caspase 9. All three pathways upregulate expression of the antiapoptotic proteins Bcl-2 and Bcl-X L . Finally, both the Jak/Stat and MapK/Erk pathways have been implicated in resistance to ROS. Thus, neurotrophic factors have the potential to attack ALS on a number of fronts.
Recombinant neurotrophic factors have been evaluated in ALS patients. However, the results of these trials have been generally disappointing, with little efficacy shown. There are many potential causes for these failures, including subtherapeutic dosing, poor penetration into the CNS, and off-target effects. It is hoped that gene therapy approaches can overcome some or all of these hurdles.
Cardiotrophin-1
Cardiotrophin-1 (CT-1) is a member of the IL-6 family of cytokines which also includes leukemia inhibitory factor and ciliary neurotrophic factor (CNTF). These cytokines have been shown to have a potent neuroprotective effect in a variety of models, including cultured primary MNs, peripheral nerve axotomy, and mouse models of neurodegeneration.
One gene therapy study has investigated the use of intramuscular injection of an Ad virus expressing CT-1. Neonatal SOD1 mice received bilateral injections of vector into the gastrocnemii, triceps brachii, and the long dorsal trunk muscles. Treatment delayed the onset of symptoms by 27 days and increased survival by 13 days. Neuromuscular function was improved, and there was both a reduction in the loss of muscle mass and improved muscle morphology. The authors hypothesized that the observed efficacy might have been achieved by direct protection of the NMJ and/or by myotrophic affects on the skeletal muscle.
Although these molecules have been shown to be effective in animal models, their translation into the clinic has not been smooth. Chronic systemic infusion of CNTF had significant side effects in clinical trials, including activation of acute-phase proteins and elevated body temperature, anorexia, weight loss, and cough. These side effects were severe enough to be dose-limiting in about half of the patients. Critically, the side effects limited the doses tested in these clinical trials to levels well below those evaluated in an animal model. Thus, it might not be surprising that a double-blind trial saw no efficacy. The possible side effects of these cytokines highlight the need to efficiently deliver the therapeutic to the spinal cord while minimizing exposure to the rest of the body. This is especially true for gene therapy approaches where discontinuing therapy may not be possible in the face of severe side effects. Employing fusion proteins to help target these cytokines to particular cell types may be one approach to consider.
Granulocyte-Colony Stimulating Factor
G-CSF was originally described as a factor that could induce the differentiation of a myelomonocytic leukemic cell line into granulocytes. While it has found many uses in the clinic targeting the hematopoietic compartment, this molecule also has roles in other tissues as well. G-CSF has been shown to be neuroprotective in multiple models. For instance, it can block glutamate-induced death in cultured neurons, and it can reduce the damage caused by stroke.
The action of G-CSF in the CNS likely goes beyond direct effects on neurons. Yamasaki et al. investigated the effect that hypoglossal axotomy had on neuron death in wild-type and SOD1 mice. The procedure was performed at 12 weeks of age, well before the animals exhibited symptoms of ALS. Unsurprisingly, 40 days after hypoglossal axotomy, neuron death was higher in SOD1 mice than in wild-type controls. However, in the time frame of 3–20 days postaxotomy, staining for Iba1 showed more microglia in wild-type mice than in SOD1 mice, suggesting a protective role for microglia. These glia expressed the G-CSF receptor and were GDNF-positive. Treatment of these mice with G-CSF further increased the number and size of the microglia. Since the effect of G-CSF on microglia appeared to be neuroprotective, the authors evaluated recombinant G-CSF as a therapy for ALS.
In SOD1 mice subcutaneous injection of G-CSF (100 µg/kg per day, 5 days a week) was initiated at 10 weeks of age. This therapy increased life span by 8 days. MN survival at the early symptomatic phase of the disease was not improved, but there was an increase in the number of large myelinated axons in the C5 ventral root. In vitro, microglia and peritoneal macrophages from SOD1 animals exhibited less migration when stimulated with monocyte chemotactic protein-1 compared to cells from wild-type animals. Looking for the basis for this finding, the authors found reduced levels of matrix metalloproteinase-9 in SOD1 microglia, an enzyme needed for transmigration. In addition, there were reduced levels of both phosphorylated p38 MAPK and NF-κB p65, suggesting that impaired signal transduction underlies the migration defect. Treatment of SOD1 microglia with G-CSF resulted in the nuclear translocation of phosphorylated Stat3 and significantly improved the ability of the cells to migrate.
Given that chronic dosing will be required for ALS therapies and that G-CSF has functions outside the CNS, Henriques et al. investigated a CNS-directed gene therapy approach. AAV1-G-CSF was delivered by intraspinal injection into 10-week-old SOD1 mice. This vector produced high levels of G-CSF in the cord, but only mildly elevated levels in the serum. Neutrophil counts were elevated by threefold, but this was still in the normal range. Disease onset, as measured by a loss in body weight, was delayed by 2 weeks. MN counts and motor function showed some improvement, and NMJ innervation was improved by about 50%. Life span was increased by 15 days. Interestingly, microglial activation seemed unchanged, a deviation from that which was observed in the axotomy study.
Vascular Endothelial Growth Factor
As the name implies, VEGF was originally discovered due to its ability to serve as a mitogen for vascular endothelial cells. In the intervening years, it has been discovered that the first protein discovered, now termed VEGF-A, is only one member in a family of similar factors. The other members are VEGF-B, -C, -D, -E, -F, and placental growth factor. Since VEGF-A is the only member that has been investigated as a therapeutic for ALS, this chapter will focus specifically on this gene and simply refer to it as VEGF. VEGF transcripts can undergo alternative splicing, yielding six proteins designated by their amino acid length: VEGF-121, VEGF-145, VEGF-165, VEGF-183, VEGF-189, and VEGF-206. The majority of VEGF produced is generally the VEGF-165 form, and the majority of ALS studies employing VEGF use this isoform.
In addition to its role in angiogenesis, VEGF plays important roles in the CNS as well. It can stimulate neurogenesis and is involved in axon guidance. VEGF is also neuroprotective in the face of a number of insults that may play a role in ALS. By activating both the ERK and PI3K/Akt pathways, VEGF significantly inhibits glutamate-induced cell death. This effect may be due to the inhibition of excitotoxic processes rather than to caspase-dependent apoptosis. VEGF also protects neurons from hypoxia via signaling through the VEGFR-2 receptor and the PI3K/Akt pathway. It may also play a role in inhibiting apoptosis.
A lot of the enthusiasm for VEGF as a therapeutic agent for ALS began with the study of a mouse knock-out of the hypoxia-response element in the VEGF promoter. This knockout has reduced expression of VEGF, primarily in neural tissue. Although 60% of knockouts die before or around birth, the remainder survive more than 23 months. The survivors develop significant muscle weakness by 5 months of age. Sensory systems, though, are not affected. Muscles atrophy, motor unit action potentials are significantly reduced, and the latency of compound muscle action potentials (CMAPs) is reduced in these mice. MN loss is detectable by 7 months of age, and 30% of MNs are lost by 17 months of age. Reactive astrocytosis is evident in the ventral horns, and phosphorylated neurofilaments are observed in older knock-out mice. Thus, these mice recapitulate many of the pathologic and behavioral aspects of ALS. Interestingly, resting neural blood flow rates are reduced by about 40% in the knock-out mice, while blood flow in other tissues is unaffected, supporting a role for hypoxia in the development of ALS.
There have been a number of proof-of-principle studies examining the effects of VEGF on mutant SOD1. Using NSC34 cells transduced with an Ad vector expressing wild-type or mutant (G93A) SOD1, Li et al. showed that overexpression of mutant SOD1 increased oxidative stress and decreased cell viability. Pretreatment of cells with VEGF was effective at reducing the cytotoxic effects of mutant SOD1 expression. Since VEGF can signal through PI3K/Akt and MAPK, the contribution of these two pathways on the phenotype was examined. In an elegant series of experiments, they showed that PI3K was necessary to transmit the effect of VEGF. The authors hypothesized that Akt might inhibit apoptosis by inactivating glycogen synthase kinase 3, forkhead transcription factors, and/or caspase 9.
Moving to an in vivo model, Wang et al. crossed a transgenic mouse that expressed VEGF-165 from the NSE promoter with the SOD1 mouse to see whether neuronal expression of VEGF-165 could alter the disease state. VEGF;SOD1 double transgenics had significantly reduced MN loss. Disease onset was delayed by 20 days. However, disease progression after the onset of motor deficits was similar to that of SOD1 mice. Thus, life span was similarly increased by 23 days. This demonstrated that neuronal expression of VEGF-165 can be protective in ALS.
Two studies have examined the efficacy of recombinant VEGF in SOD1 rodent models. In the first study, female SOD1 mice received either 1 µg/kg or 0.1 µg/kg of murine VEGF once a week via an intraperitoneal injection. In the high-dose group, disease onset was delayed by ~12 days, while the low dose had no discernible effect. Recognizing that continuous delivery of VEGF to the CNS might be more effective than daily or weekly systemic delivery, Storkebaum et al. evaluated recombinant VEGF delivered intracerebroventricularly by a micropump. Starting at 60 days of age, they infused 0.2 µg/kg per day of VEGF-165. Life span was increased by 22 days, and disease onset was delayed by 17 days. At 110 days of age, MN survival was substantially greater in the brainstem and cervical spinal cord in the treated mice. Rotarod performance was also significantly improved, and NMJ enervation was doubled relative to controls. Delaying treatment until symptom onset reduced the efficacy of the therapy, increasing survival by only 10 days.
Two studies have evaluated gene therapies for ALS by delivering VEGF-165. Azzouz et al. employed a rabies-G pseudotyped equine infectious anemia virus (EIAV) injected at either 21 days or 90 days, the latter corresponding to disease onset. Injection of the vector into the gastrocnemius, diaphragm, intercostal, facial, and tongue muscles at 21 days of age resulted in extensive transgene expression in the brainstem and spinal cord. Disease onset was delayed by 28 days and survival was increased by 38 days. MN survival was higher in EIAV-VEGF-treated mice at both 115 days and endpoint, although the increase at endpoint was smaller. Motor function was also improved. Similar to the use of recombinant VEGF-165, delivering the therapy at disease onset halved the improvement in life span with treated mice living an extra 19 days relative to controls. The authors noted that transduction was lower at 90 days which might be attributed to the death of MNs by that time. Since therapies for ALS will be initiated after disease onset for the vast majority of patients for the foreseeable future, this result suggests that delivery methods requiring retrograde transport of the vector will be less than ideal.
A second approach examined was to use AAV4 to express VEGF-165 in choroid plexus epithelial cells and ependymal cells of the ventricles. The hope was that these cells would secrete the protein into the cerebrospinal fluid where it could be delivered throughout the CNS. SOD1 mice received bilateral intracerebroventricular injections at 85 days of age. Due to sex-related differences in disease presentation, males and females were analyzed separately. AAV4-treated female SOD1 mice had a 20-day increase in life span relative to controls, while the life span of males increased by 9 days. Measurements of motor function showed improvement for both sexes.
As previously described, the VEGF gene produces at least six different isoforms, and studies have suggested that expressing combinations of these isoforms is more efficacious than expressing single isoforms. This provides a challenge for current gene-therapy vectors, since their DNA and RNA packaging limits are generally large enough for cDNAs but insufficient to package entire genes. How might one then tackle this challenge for VEGF expression? One approach, taken first by Rebar et al., is to engineer a zinc finger protein (ZFP) transcription factor to increase VEGF expression from the endogenous gene. This approach has the benefit of increasing the expression levels of the individual isoforms while preserving their expression levels relative to one another.
Sakowski et al. studied the use of this transcription factor in rats that underwent a recurrent laryngeal nerve crush injury that causes vocal cord paralysis. Delivery of an adenovirus expressing the transcription factor immediately after the nerve crush injury significantly improved recovery of vocal cord function, suggesting that this approach might be useful for treating diseases that exhibit loss of innervation by MNs.
Kleim et al. attempted to extend this work to ALS. 80-day-old rats received weekly injections of a plasmid expressing the ZFP transcription factor unilaterally into the medial gastrocnemius muscle for six weeks. No significant improvements were observed for MN survival, NMJ innervation, and muscle fiber composition, but there was a hint of some improvement observed in hindlimb grip strength and rotarod performance. The authors did not report any data on transgene expression. However, it is unlikely that they succeeded in transfecting MNs, and expression in the muscle was probably suboptimal relative to what could be achieved using viral vectors. It would be interesting to see how effective this approach would be using more efficient delivery methods.
Brain-Derived Neurotrophic Factor
BDNF is a member of the neurotrophin family, and it is expressed throughout the CNS. Like other neurotrophic factors, BDNF can reduce or prevent neuronal death caused by a wide variety of insults. These include many possible contributors to ALS, such as glutamate toxicity, toxic proteins, and hypoxia. In addition, BDNF can also affect synapses, increasing axonal branching, which could be beneficial at the NMJ. One gene therapy study examined intramuscular injection of naked DNA expressing BDNF. No significant improvements in life span or motor function were found, but caspase activation was reduced.
Some have argued that BDNF is a poor choice for the protection of MNs. In vitro BDNF not only fails to protect MNs from glutamate-induced cytotoxicity, but it increases sensitivity through activation of the PI3K signaling pathway. A lack of protection against cytotoxicity was also found in vivo. Another potential hurdle is the MN itself. This cell type expresses a truncated form of the BDNF receptor, TrkB, that appears to reduce the efficacy of BDNF. These findings suggest that BDNF alone will not be therapeutic.
Glial-Derived Neurotrophic Factor
GDNF is a neurotrophic factor that expressed in both CNS and non-CNS tissues. Those locations that are likely relevant for ALS include the spinal cord, Schwann cells, and skeletal muscle. In the hope of identifying a therapeutic molecule for Parkinson’s disease, GDNF was originally described as a neurotrophic factor that could protect midbrain dopaminergic neurons in cell culture. In vitro it increased the size of dopaminergic neurons and enhanced neurite outgrowth. In vivo, GDNF could protect these neurons in a chemically induced model of Parkinson’s disease.
Could GDNF be neuroprotective for MNs as well? Interestingly, activation of GDNF’s receptor, RET, is reduced in the MNs of ALS patients, suggesting that reduced GDNF signaling may contribute to disease progression. GDNF is a potent protector of cultured embryonic MNs and can protect MNs from serum deprivation. In vivo it can protect fetal MNs from the usual apoptosis that occurs during development and from a number of insults, including excitotoxicity.
A number of studies have evaluated delivery of GDNF to the muscle. Manabe et al. injected recombinant GDNF unilaterally into the gastrocnemius muscle of SOD1 mice three times a week beginning at 35 weeks of age. At 46 weeks of age, MN counts were higher in GDNF-treated SOD1 mice on the injected side, and there was a similar increase in the number of phosphorylated Akt + MNs. However, the activation of caspase 3 and caspase 9 was not suppressed, and phosphorylated ERK staining was unchanged. Ciriza et al. injected GDNF fused to the tetanus toxin heavy chain C fragment (TTC) into each limb beginning at disease onset. This extended life span by 8 days and improved performance in an open-field test.
To avoid the need for repeated injection of GDNF, Mohajeri et al. created myoblasts overexpressing GDNF using retroviral vectors. These cells were transplanted bilaterally into the medial and lateral gastrocnemius muscles of 6-week old SOD1 mice. This led to increased survival of large-diameter MNs, delayed disease onset and loss of motor function, and slowed muscle atrophy.
Transducing the muscle directly may be preferable to ex vivo transduction of myocytes. Injection of an AAV2 vector expressing GDNF into the gastrocnemius muscles led to transduction of 30% of the muscle fibers. GDNF levels increased over the first 2 months and then remained fairly stable for at least 10 months. GDNF was concentrated in the sarcolemma, suggesting that the protein was secreted and concentrated at NMJs. GDNF was also retrogradely transported from the muscle to the cell bodies of MNs.
To determine how this therapy impacts disease onset and progression, SOD1 animals were injected at 9 weeks of age into the gastrocnemius and triceps brachii muscles. Expression of GDNF was about 120-times normal. At 110 days of age, muscle atrophy was reduced by about 75%. Treatment preserved ~40% of the MNs that would have died by this time point. Rotorod testing showed improved motor function. Disease onset was delayed by 13 days, and survival was increased by 17 days.
In a test of nonviral gene therapy, Yamamoto et al. electroporated a GDNF plasmid into tibialis anterior and gastrocnemius every 2 weeks beginning at 9 weeks of age. GDNF was present in both the muscle and MNs. Survival was not improved, but motor function did respond to therapy. Moreno-Igoa extended this line of study by delivering the therapeutic plasmid to both the forelimbs and hindlimbs. The plasmid was injected into the quadriceps and triceps of SOD1 mice at 8 weeks of age. Disease onset was delayed by 9 days and life span was increased by 15 days. Motor function was also improved relative to control mice. To begin to understand what aspects of the disease GDNF was targeting, the authors evaluated a number of molecular markers. Caspase-3 activation was reduced to levels similar to that of wild-type mice. Bax and Bcl2, both upregulated in SOD1 mice, were reduced. Phosphorylated Akt was elevated in treated mice, suggesting activation of survival signals. In SOD1 mice there was increased phosphorylation of ERK1/2; GDNF reduced this to almost normal levels.
A few studies have targeted GDNF expression to the CNS. Foust et al. injected AAV5-GDNF into the red nucleus and motor cortex to get anterograde transport of GDNF to the spinal cord via the rubrospinal tract (RST) and the corticospinal tract, respectively. The RST path delivered more GDNF to the spinal cord. GDNF concentration dropped with increasing distance from the brain, which could have implications for clinical translation. However, no therapeutic application was measured in this study.
Guillot et al. looked at the efficacy of lentiviral delivery of GDNF in SOD1 mice. Intraspinal injections were performed in 40-day-old SOD1 mice. Onset of disease and MN death was not affected. In contrast, when the vector was delivered to the facial nucleus, the therapy protected about 1/3 of the MNs that would have died 3 months after injection. The difference in efficacy for these two cell populations might suggest that there are differences in how these different populations respond to GDNF. Alternatively, it may simply reflect differences in disease severity, as MN loss is slower in the facial nucleus.
Similar results were found when human cortical neural progenitor cells were transduced with a lentivirus expressing GDNF and transplanted into the spinal cord. There was wide distribution of transplanted cells throughout the lumbar cord on the injected side and high levels of GDNF expression. MN loss was essentially prevented on the injected side, but the therapy failed to preserve innervation of NMJs. Consequently, no improvement in limb function was observed.
The CNS-directed studies suggest that delivery of GDNF solely to the CNS will not be effective for the treatment of ALS. Instead, a GDNF approach may need to target both the MN soma and the NMJ. Ad vectors have the potential to transduce both MNs and muscle when injected intramuscularly. Acsadi et al. performed bilateral injections of Ad-GDNF into the anterior tibialis, gastrocnemius, quadriceps, and paraspinal muscles of neonatal SOD1 mice. Injecting neonates avoided the immune response that normally plagues Ad-mediated gene therapies. Expression was stable from 1 to 4 months in the muscle and present in the spinal cord for at least 4 months, although 72% of the expression there was lost over time. Disease onset was delayed by 9 days, and life span was increased by 13 days. Treatment improved rotarod performance and slowed MN loss.
An attempt to initiate therapy closer to the time of disease onset failed to significantly alter the disease trajectory. Due to the immunogenic nature of Ad, this therapy had to be given weekly when initiated in adult animals. Because of the immune complications, the results of this study may not be representative of the potential for combined CNS/muscle delivery of GDNF. However, as studies of VEGF have suggested, loss of innervation at disease onset may also make retrograde transport-dependent therapies difficult to translate.
Insulin-Like Growth Factor 1
IGF-1 was discovered far from the brain. This neurotrophic factor was originally identified as a mediator for the effects of the somatotrophic hormone. Since that initial discovery, researchers have learned that the roles that this molecule plays go far beyond skeletal growth. IGF-1 and its receptor are expressed in several regions of the CNS, including on MNs. When overexpressed in muscle, IGF-1 can accelerate the recovery of function following a nerve crush injury. It can also block the death of MNs in vitro, inhibit MN death in embryos following axotomy, and protect against excitotoxicity. Thus, IGF-1 has been a strong candidate for ALS therapies.
Kaspar et al. investigated retrograde delivery of an AAV vector expressing IGF-1, comparing its efficacy with that of GDNF. When given at 60 days of age, IGF-1 increased median life span by 37 days, while GDNF extended life span by only 11 days. When given at disease onset, IGF-1 therapy increased median life span by 22 days, while GDNF extended it by 7 days. IGF-1 treatment delayed weight loss, improved motor function, reduced cellular vacuolization, and reduced MN loss. Astrogliosis was also reduced. Ubiquitin-positive aggregates in the spinal cord were ameliorated. TUNEL staining suggested that apoptosis was reduced as well. Using a lentivirus to deliver IGF-1 expression to only the muscle yielded a smaller improvement in life span (9 days), suggesting that expression of IGF-1 in MNs was critical for the therapy. Akt, a kinase activated by IGF-1, exhibited elevated phosphorylation with IGF-1 treatment. This likely led to the reduction in activation of caspases 3 and 9. Tumor necrosis factor alpha (TNF-α) was also reduced, suggesting a mechanism by which IGF-1 dampened microgliosis and astrogliosis. In unpublished data, the authors noted that ubiquitin-positive aggregates were smaller and more focalized in IGF-1-treated mice, suggesting that the therapy also enhanced degradation of the aggregates or slowed their production.
Two studies evaluated parenchymal injection of AAV2-IGF-1 into the spinal cord. The first evaluated delivery of AAV2-IGF-1 into the lumbar cord of 60-day-old, presymptomatic SOD1 mice. This route resulted in neuronal transduction. MN survival was higher in IGF-1-treated mice at 110 days of age, but substantially lower than wild type. Microgliosis was unaffected. In males, disease onset was delayed, but once it started, the rate of motor function loss was similar to that of control animals. Survival in males was increased by 12 days. No improvement was observed in SOD1 females. Franz et al. found similar results following unilateral parenchymal injection into the cervical spine.
Dodge et al. have undertaken two approaches to try to deliver IGF-1 to a broader section of the CNS. In their first study, AAV1-IGF-1 was injected into the deep cerebellar nuclei in SOD1 mice at ~89 days of age. This site was chosen because it has multiple afferent and efferent connections to all levels of the spinal cord. The authors hoped that this would translate to vector delivery along the length of the cord via retrograde transport. Improvement was seen in MN survival at 110 days of age. Grip strength and rotarod performance was improved relative to mock-treated controls, and life span was increased by 14 days. Astrogliosis and microgliosis were dramatically reduced as were nitric oxide synthase and 3-nitrotyrosine. In vitro data suggested that IGF-1 acted both through MNs and through modulation of glial activation. In their second study, Dodge et al. used an AAV4 to drive secretion of IGF-1 from the choroid plexus and ependymal cells as they did with VEGF. SOD1 mice received bilateral ICV injections at 85 days of age. Improvements were seen in survival and motor function.
Like VEGF, IGF-1 can also generate an array of isoforms with distinct functions. IGF-1 has two major isoforms that arise from alternate splicing: circulating (class 2) and local (class 1). The former is expressed in the liver and is secreted. The latter is expressed in other tissues and generally stays within the tissue. One study crossed a transgenic mouse with muscle expression of the local isoform of IGF-1 (mIGF-1) with a SOD1 mouse. mIGF-1;SOD1 mice exhibited a delay in disease onset of 10 days and an increase in survival of 30 days. Muscle atrophy was attenuated, and markers of satellite cell activity suggested that these cells contributed to muscle maintenance. The mIGF-1;SOD1 mice also maintained fiber composition longer. NMJs showed more innervation in mIGF-1;SOD1 mice than in SOD1 mice, even at end-stage disease. MN survival was increased relative to SOD1 mice, and astrogliosis was delayed. The authors also looked for evidence of muscle regeneration. They had previously shown that the calcineurin-A mRNA was alternatively spliced during muscle regeneration. Although SOD1 mice and mIGF-1 transgenics did not express this isoform, mIGF-1;SOD1 mice expressed this protein after the onset of clinical symptoms. This could point to a mechanism by which IGF-1 could prolong muscle integrity after denervation began.
Hepatocyte Growth Factor
Hepatocyte growth factor (HGF) was originally identified by its effects outside of the CNS, functioning as a mitogen for mature hepatocytes. Like many signaling molecules, though, this factor plays roles in a variety of tissues, including the nervous system. HGF and its receptor, Met, are expressed in the brain in both neuronal and nonneuronal cells. During development, HGF is necessary for MN neurite outgrowth to the limb, serving as a chemoattractant for MNs. In vitro HGF promotes the survival of MNs, while in vivo it protects them from axotomy. In SOD1 rats, HGF and Met levels increase with disease progression, possibly pointing to a protective role for HGF signaling in ALS.
To determine whether this growth factor might be therapeutic for ALS, Sun et al. created a transgenic mouse overexpressing rat HGF in the CNS using the NSE promoter. When crossed to the SOD1 mouse, this transgene delayed MN loss by more than a month and protected axons in the ventral roots. HGF;SOD1 animals also had improved motor function, and median survival was increased from 260 days to 287 days. Interestingly, the effect of HGF did not appear to be through the induction of the antiapoptotic gene Bcl-X L . However, HGF did reduce induction of caspase-1 and iNOS. When studying astrocytes in HGF;SOD1 mice, they found that astrogliosis was attenuated and EAAT2 levels were substantially elevated.
Ishigaki et al. studied the effects of continuous intrathecal administration of recombinant human HGF to SOD1 rats. MN death and disease onset were delayed in a dose-dependent manner. When given just before disease onset, the highest dose, 100 µg/week, increased survival by 11 days, and the decline in motor function was slowed relative to controls. There was a concomitant delay in MN loss 2 weeks after therapy was begun. Phosphorylated c-Met was increased in MNs in the treatment group, and activated caspase-3 and caspase-9 were reduced relative to the control group. By western blot, EAAT2 was elevated with treatment, but there was no significant change in GFAP expression. While gene therapy using HGF has not yet been evaluated, the above studies suggest that it might generate effects similar to those of other neurotrophic factors.
Combination Therapies
With such a broad array of neurotrophic factors to choose from, one might wonder whether combining two or more might lead to increased efficacy. A handful of studies have begun to address this question. Their results suggest that choice of which factors to pair together will be critical in determining whether two factors are better than one.
Dodge et al. combined their IGF-1 and VEGF-165 vectors targeting the choroid plexus and ependymal cell layer. While both had similar effects on alleviating the disease, the combination of the two was no better than the individual factors. It has been hypothesized that the effects of IGF-1 might be mediated by VEGF. This could explain the lack of benefit from the combined therapy.
Haase et al. investigated intramuscular injection of Ad-neurotrophin-3 (NT-3) and/or Ad-CNTF into neonatal progressive motor neuronopathy ( pmn ) mice. This model exhibits muscle paralysis beginning at 2 weeks of age and death at 6–7 weeks. All three treatments (NT-3 alone, CNTF alone, or the combination of the two) increased median life span by about 21 days. Compound muscle action potential amplitudes were higher in treated mice (40% vs 70% of normal). Spike amplitudes increased in treated mice to levels higher than those of wild-type and pmn mice, suggesting that these neurotrophic factors could induce peripheral or collateral sprouting. Indeed, examination of muscle showed that Ad-NT-3 and the combination therapy resulted in substantially increased branching of axons that succeeded in reinnervating NMJs that were not innervated in untreated animals. Treatment reduced axonal degeneration in the phrenic nerve fibers. Combining Ad-NT-3 with Ad-CNTF did not further increase life span, but did improve MN counts and the number of myelinated fibers. Although this model is not necessarily a model of ALS, the increased branching driven by the NT-3 vector could be useful for restoring innervation of NMJs in ALS patients, possibly restoring some motor function.
Krakora et al. performed ex vivo gene delivery to express GDNF and/or VEGF in mesenchymal stem cells. Following the induction of muscle damage, these cells were injected into the forelimb triceps brachia, tibialis anterior, and long muscles of the dorsal trunk of SOD1 rats. The individual neurotrophic factors extended life span by 14 days. Combining the two extended life span by 28 days and did a better job preserving NMJ innervation and motor function.
Mitsumoto et al. evaluated the effects of recombinant CNTF and BDNF on the wobbler mouse. This model has a defect in a vesicle transport factor and recapitulates many of the abnormalities found in ALS. While the individual factors could merely slow disease progression, the combination of the two halted disease progression for at least 1 month. As noted earlier, there are many factors working against BDNF as a therapy for ALS. One study of BDNF in SOD1 mice failed to find efficacy, albeit with a poor delivery system. Thus, the synergy between CNTF and BDNF is quite remarkable. Neurotrophic factors rarely work in isolation from one another, and this may be a case where one factor is able to sensitize the system to the second, producing a substantially larger effect.
Combining therapies is not limited to neurotrophic factors alone. Kaspar et al. combined IGF-1 therapy with exercise. AAV2-IGF-1 was injected into the intercostal and quadriceps muscles of 90-day-old SOD1 mice. The mice were given exercise wheels at 40 days of age, with different groups being offered the wheel for different lengths of time (2 hours, 6 hours, or ad libitum ). Exercise alone led to increases in life span (7, 40, and 24 days, respectively). IGF-1 extended life span by 29 days. Combining exercise and gene therapy substantially increased median survival by 83 days.
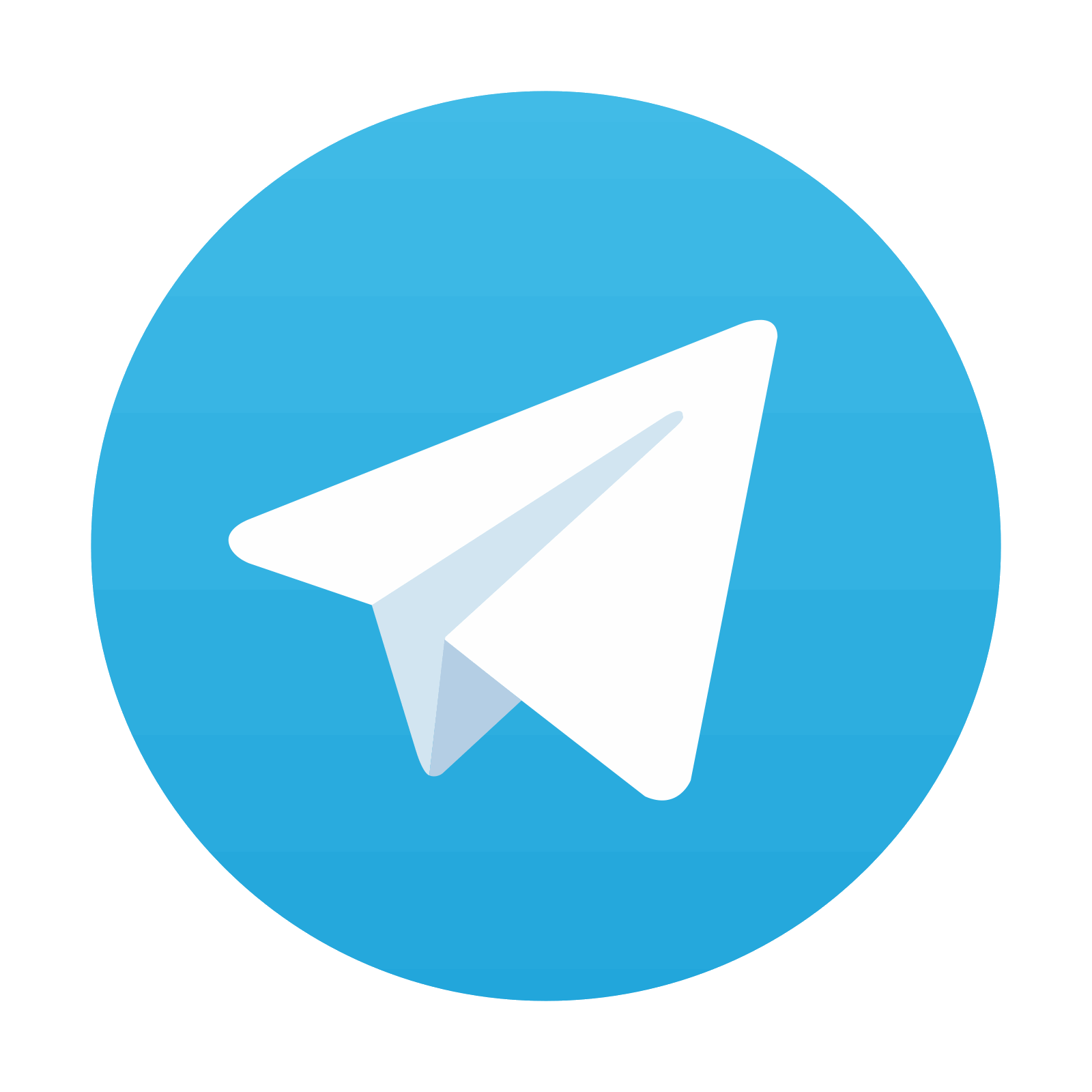
Stay updated, free articles. Join our Telegram channel
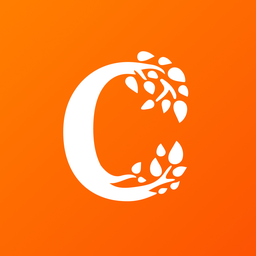
Full access? Get Clinical Tree
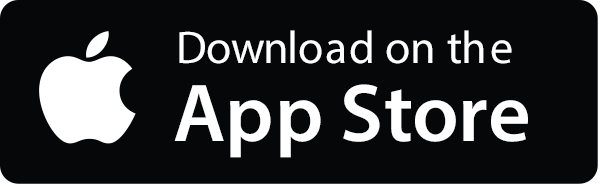
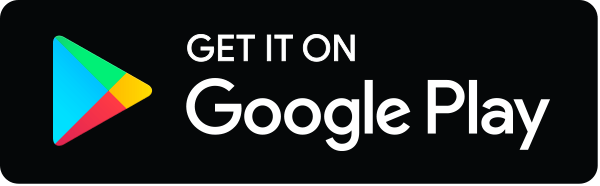